Evaluation of the infant, child, or young adult with cardiovascular symptoms may require the application of a variety of cardiac tests. Each of these tests has specific indications that provide unique information for characterization of a particular problem, but these tests also have limitations. Adding various tests allows for the layering of information until a rich composite model of the cardiovascular structure and function is built. This allows for optimal understanding of the condition and the implementation of the most appropriate and efficient care.
Electrocardiography has always been one of the fundamental procedures used in the diagnosis and treatment of cardiac disease ever since Einthoven first recorded the heart’s electrical activity in 1901 using a string galvanometer. Information gained from the electrocardiogram (ECG) can be used to help determine the cardiac anatomy, the cardiac rhythm and electrical conduction, and the effect of therapeutic interventions on cardiac electrical activity. Since the advent of echocardiography, a pediatric cardiologist rarely depends on the ECG to accurately predict the cardiac anatomy. However, the ECG is indispensable in the evaluation of a child with a suspected cardiac rhythm abnormality. It is now well recognized that some medications may significantly alter the heart’s electrical properties to the point that it places the patient at increased risk for sudden cardiac death, usually from a form of polymorphic ventricular tachycardia known as torsade de pointes. As such, screening and serial ECGs are indicated. Although still an area of controversy in the United States, there are proponents who say that an ECG should be included in the preparticipation sports evaluation and before the initiation of stimulant medication for attention-deficit hyperactivity disorder.1-4 Although today’s ECG machines are smaller, portable, and readily available, the waveforms they produce are not different than those seen by Einthoven over a century ago.
For a complete description of the ECG, the reader is referred to the many textbooks devoted exclusively to ECG interpretation. The basic 12-lead ECG includes the limb leads I, II, III, aVR, aVL, and aVF plus the precordial leads V1 to V6. Because most pediatric cardiologists see children with anatomically abnormal hearts, the typical pediatric ECG records 15 leads, the additional 3 being lead V3R, V4R, and V7 (Figure 2-1). The different leads that are used in the ECG provide unique perspectives for the evaluation of the electrical system of the heart as a whole. This concept is represented by the hexaxial reference system and the horizontal reference system (Figure 2-2). In the hexaxial system, the limb leads represent different degrees of orientation in relationship to the vertical plane of the heart from 0 to ±180 degrees. The positive or negative deflections created by the depolarization forces in these different leads may be interpreted using this vector system to identify the cumulative direction of the electrical stimulus within the myocardium. Meanwhile, the horizontal reference system represents the precordial leads V1 to V7, as well as V3R and V4R. This system provides information about the anteroposterior and left to right aspects of the horizontal plane of the heart.5 The pediatric leads V3R and V4R allow for a more thorough evaluation of the right ventricle, whereas V7 allows for a more thorough evaluation of the left ventricle.
Figure 2-2

Hexaxial and horizontal reference systems. The ECG records the net sum of the heart’s electrical activity over time. Lead placement in relation to the heart determines whether a potential difference between the heart and the electrode is a positive or negative deflection. (Reproduced, with permission, from Tintinalli JE, Stapczynski JS, Ma OJ, Cline DM, Cydulka RK, Meckler GD. Tintinalli’s Emergency Medicine: A Comprehensive Study Guide. 7th ed. New York, NY: McGraw-Hill; 2011.)
As with all parameters in pediatrics, the ECG also has a wandering baseline of normal for nearly all measurements. One must know the age and the clinical condition of the patient before any meaningful interpretation of the ECG can be made. Davignon’s published normative data by age in 1979 is still used, but many institutions have subsequently developed their own standards.6 A systematic approach to ECG interpretation is recommended. Heart rate; QRS and P-wave axis; PR, QRS, and corrected QT intervals; evidence of right or left ventricular hypertrophy; ST and T-wave patterns; and assessment for cardiac rhythm abnormalities should be evaluated sequentially. Some ECG machines have an automated interpretation function that should only be used as a preliminary read of the study.
Average heart rates at rest should generally be below 100 beats per minute (bpm) after age 5 years. Neonatal heart rates above 235 bpm are usually not secondary to sinus tachycardia but are more typical of a true cardiac tachydysrhythmia. Similarly, a 6-year-old with a fever and a heart rate of 130 bpm may simply have sinus tachycardia secondary to the fever with no evidence of cardiac disease. Again, the age and clinical condition of the child must be known to accurately interpret the ECG.
The QRS axis is measured using leads I and aVF. It is a limited element of the ECG that can assist in the diagnosis of congenital heart disease. Typically, newborns have a mean QRS axis that is rightward, negative in lead I and positive in lead aVF. The mean QRS axis drifts leftward after birth generally assuming the normal adult QRS axis of 0 to 90 degrees by 1 month of age. Children with trisomy 21 associated with a complete common atrioventricular (AV) canal cardiac defect usually have an ECG that demonstrates a superior mean QRS axis. In leads I and aVF, the QRS is negative, whereas in leads aVR and aVL, it is positive. It must be remembered, however, that all children with Down syndrome should have a formal cardiac evaluation and echocardiogram performed in the neonatal period, even if the ECG is normal. Children with tricuspid atresia may have cyanosis and an ECG with left axis deviation. Right axis deviation with right ventricular hypertrophy can be seen in children with pulmonary hypertension. Most types of congenital cardiac defects do not have a unique QRS axis, but an abnormal QRS axis for age should raise the possibility that a cardiac abnormality may be present.
Measurement of the P-wave axis is performed using the same leads as the measurement of the QRS axis. In a normal heart, it falls between 0 and 90 degrees, indicating that the atrial activity is originating in the high right atrium, the location of the sinus node. A P-wave axis outside of that quadrant is consistent with an ectopic atrial rhythm, limb lead reversal, or dextrocardia. P and QRS right axis deviation associated with decreasing QRS amplitudes from V1 to V6 is strongly suggestive of mirror-image dextrocardia. These are minor points, but it is something a cardiologist looks for in a child with a murmur or history of palpitations.
Variations in the PR interval are typically seen in healthy, athletic adolescents, especially during sleep. The pattern seen varies from isolated prolonged PR intervals known as first-degree AV block, more accurately described as prolonged AV conduction, to Mobitz type I, second-degree AV block with progressively lengthening PR intervals, also known as Wenckebach phenomenon. With Wenckebach phenomenon, there is progressive prolongation of the PR interval until there is a loss of conduction to the ventricle. The cause is usually increased vagal tone. More pathologic manifestations of AV nodal conduction occur with Mobitz type II, second-degree AV block when the PR interval stays constant but P waves are conducted to the ventricle in various conduction ratios such as 2:1, 3:1, and 4:1. This condition may progress to complete heart block, in which the P waves and QRS complexes are completely dissociated from one another. PR prolongation may also be seen after ingesting drugs that affect AV conduction or as an effect of increased intracranial pressure, such as an obstructed central nervous system shunt used in hydrocephalus. Conversely, short PR intervals are seen in patients with Wolff-Parkinson-White syndrome due to pre-excitation of the ventricle through an accessory pathway. The ECG in Pompe disease characteristically demonstrates a short PR interval associated with biventricular hypertrophy.
The QRS duration varies with age. In most children, it is less than 90 ms. In infants, it is somewhat less than that. This is important because in the neonatal period, ventricular tachycardia may be mistaken for supraventricular tachycardia. The location of open heart surgery increases the risk of either right bundle-branch block or left bundle-branch block, both having a characteristic wide QRS pattern. Surgery that involves closure of a ventricular septal defect, such as in tetralogy of Fallot, may injure the right bundle branch of the conduction system, which results in a right bundle-branch block pattern. Left ventricular outflow tract surgery may injure the left bundle branch, causing left bundle-branch block. Other causes of a wide QRS interval are a premature ventricular depolarization, a premature atrial depolarization with aberrant conduction, a ventricular paced depolarization, and ventricular pre-excitation as seen in Wolff-Parkinson-White syndrome. Certain antiarrhythmic medications and hyperkalemia may prolong the QRS duration. Dilated cardiomyopathy, arrhythmogenic right ventricular dysplasia, and Kearns-Sayre syndrome may demonstrate wide QRS patterns.
Measurement of the corrected QT interval is important for any child presenting with syncope or a seizure. Long QT syndrome is a cardiac repolarization abnormality that makes the heart vulnerable to a form of polymorphic ventricular tachycardia known as torsade de pointes. In some cases, the ventricular tachycardia degenerates into ventricular fibrillation and death. Measurement of the corrected QT interval must be made on a clean, high-quality original or photocopied ECG using the Bazett formula: QT corrected = QT interval Ă· Preceding R-R interval. The proper measurements cannot be made from a faxed copy of the ECG. The upper limit of normal varies among cardiologists, but 460 ms is probably a reasonable number.7 If a patient has a history of dizziness, palpitations, syncope, or seizures and a prolonged corrected QT interval, consultation with a cardiologist is recommended. There is a growing list of medications that may cause prolongation of the corrected QT interval. This information is readily available from a pharmacist or hospital formulary and available online at www.qtdrugs.org.8
Before echocardiography, cardiac chamber enlargement and hypertrophy were routinely assessed by ECG. Tall, pointed P waves in lead II suggested right atrial enlargement, or P pulmonale, and biphasic P waves in lead V1 suggested left atrial enlargement, or P mitrale. Similarly, there are many different rules for determining the presence or absence of left and right hypertrophy based on ECG findings. Unfortunately, in children with structurally abnormal hearts, some of the rules do not apply. The same can occur in children with chest wall deformities such as pectus excavatum or carinatum and scoliosis. It is not uncommon to see an ECG pattern of left ventricular hypertrophy in a healthy, lean child only to find out that an echocardiogram demonstrates no hypertrophy. An R wave greater than 30 mm in V1 is a good marker of probable right ventricular hypertrophy in a child greater than 4 years old. A Q wave in V1 also suggests right ventricular hypertrophy, in addition to a prominent S wave in V6 greater than 5 mm in patients over 1 year of age. In the pediatric ECG, the T wave in V1 is upright immediately after birth but subsequently becomes inverted within the first week of life until adolescence. An upright T wave in V1 from 1 week of age to the beginning of puberty suggests right ventricular hypertrophy. R waves greater than 25 mm in V6, Q waves greater than 5 mm in V6, and S waves greater that 20 mm in V1 suggest left ventricular hypertrophy.
ST-segment abnormalities and T-wave inversions are commonly seen in adult medicine in the setting of ischemia and infarction of the myocardium secondary to coronary artery disease. Compromise to coronary perfusion is much less common in the pediatric population but can occur in certain settings. During ischemia, T waves become inverted. ST-segment elevations develop with acute infarction of the myocardium, and Q waves develop with tissue necrosis.9 The inferior leads II, III, and aVF represent the inferior region of the myocardium perfused by the right coronary artery. Leads V1 to V4 represent the anterior region of the myocardium supplied by the left anterior descending artery, whereas leads I, aVL, V5, and V6 represent the lateral region of the heart supplied by the circumflex artery. In patients with dextro-transposition of the great arteries, a perfusion defect may develop after the arterial switch operation due to complications from the transfer of the coronary arteries. Cases of anomalous origin of the coronary arteries may also produce ST and T-wave changes, as well as prominent Q waves, whereas Prinzmetal angina may show abnormalities secondary to coronary vasospasm. Thrombi compromising the coronary circulation can occur with aneurysms of the coronary arteries secondary to Kawasaki disease.
If there are diffuse ST-segment elevations that do not follow a pattern of coronary ischemia, pericarditis should be considered. In addition, early repolarization, also known as J point elevation, is a type of ST-segment elevation pattern that is a variant of normal. An ECG with a right bundle-branch block pattern with ST-segment elevations in V1 to V3 suggests Brugada syndrome, an inherited disease caused by a mutation in cardiac sodium channels that places the patient at risk for sudden cardiac death (Figure 2-3). A pattern of diffuse T-wave inversions with signs of left ventricular hypertrophy is commonly seen in patients with hypertrophic cardiomyopathy.
The 15-lead ECG with a rhythm strip is the test of choice for documenting and diagnosing cardiac rhythm abnormalities if the cardiac dysrhythmia is occurring at the time the tracing is being obtained. Premature atrial and ventricular depolarizations, supraventricular and ventricular tachydysrhythmias, and all forms of heart block can be readily identified. In a person with a history of supraventricular tachycardia, the characteristic short PR interval with a delta wave denoting ventricular pre-excitation or Wolf-Parkinson-White syndrome can be seen during sinus rhythm (Figure 2-4). Long rhythm strips recording the lead with the clearest P wave can be very helpful when interpreting complex dysrhythmias.
Figure 2-4

Wolff-Parkinson-White (WPW) syndrome. This tracing shows evidence of an accessory pathway that is seen in patients with WPW. The PR interval is shortened (double arrow) and a delta wave (upsloping initial QRS segment) is seen (arrow, shaded area). (Reproduced, with permission, from Koop KJ, Stack LB, Storrow AB, Thurman RJ. The Atlas of Emergency Medicine. 3rd ed. New York, NY: McGraw-Hill; 2009.)
For patients who have daily episodes of palpitations or other suspected cardiac rhythm problems intermittently throughout the day, a 24- to 48-hour ambulatory ECG should be considered. Norman Jefferis Holter developed the technology in 1949. The recorders are small and lightweight and can be worn inconspicuously. A 5-lead cable is attached to the chest by snap-on lead patches. Three ECG leads are monitored continuously, and every depolarization is stored on a digital chip (Figure 2-5). The patient is encouraged to keep a diary noting the time of the palpitations and any associated symptoms. During playback, an accurate correlation between the symptom and cardiac rhythm can be made. For children who are not verbal, the parent enters their observations on the diary. The only major disadvantage to the 24-hour ambulatory ECG is that the patient cannot swim or bathe during the recording period.
Patients who have intermittent episodes throughout the month are better served using one of the cardiac event monitors known as transtelephonic event recorders. These recorders are about the size of a cellular phone and come in 2 varieties: nonlooping and looping. In a nonlooping recorder, the patient places the recorder over the heart when he or she experiences palpitations. The patient-activated recorder then records for about 30 to 90 seconds storing the tracing in the unit’s memory. The patient then calls the monitoring company’s central station at his convenience and downloads the stored ECG tracing directly over the telephone. The receptionist receives the call and forwards the tracing to the physician for review (Figure 2-6). In a looping recorder, the recorder is similar to a 24-hour ambulatory ECG monitor but uses only a 2-lead cable. The unit contains a memory chip that continuously stores about 90 seconds of data. After 90 seconds, the stored data are written over with another 90 seconds of new data. The monitor is worn continuously. The patient simply presses a button when he or she experiences any symptoms. The recorder then stores the data that were recorded about 30 seconds before the button was pressed and for about 60 seconds after activation.
A nonlooping recorder should be used for patients who have episodes that last for at least 2 minutes, the time it would take to get the recorder, place it on the chest in a private location because it has to placed directly on the skin, and activate the recorder. Looping recorders are useful in patients who have very brief episodes or in cases where it would be useful to examine the cardiac rhythm just before the onset of palpitations. In both the looping and nonlooping forms, usually more than one episode can be stored in the unit’s memory. At this time, the data can only be uploaded to the central station by a landline phone.
For more rigorous, ambulatory, long-term monitoring, there are continuous monitoring systems that work in concert with a dedicated cellular phone. These systems use a 3–chest lead array that connects to a small recorder that has the capability of analyzing the data. If it detects an irregular heart rhythm, it sends the data wirelessly to a dedicated cellular phone, which, in turn, sends the data to a central receiving station. These external cardiac ambulatory telemetry (ECAT) systems operate automatically and do not need patient activation. They are most useful in patients having recurrent syncopal episodes that are believed to be secondary to a cardiac dysrhythmia and for infants or young children with a suspected, intermittent significant dysrhythmia such as supraventricular tachycardia, ventricular tachycardia, or heart block with long pauses (Figure 2-7). For them to work properly and effectively, the dedicated cellular phone must be within 90 feet of the recorder and the patient must wear the recorder continuously except when showering, bathing, or swimming.
Finally, there are insertable cardiac monitors (ICMs) that are small, Band-Aid size recorders that are placed subcutaneously on the chest over the heart. These devices can be patient activated or can be automatically triggered. When activated, data are stored in the device and interrogated noninvasively by a programmer similar to that used for pacemaker interrogation. These monitors can function for up to 3 years. They are probably most useful for patients with syncope thought to be secondary to significant but infrequent dysrhythmias. Table 2-1 summarizes the recommendations for the various cardiac rhythm recording equipment.
15-Lead Electrocardiogram | 24- to 48-Hour Ambulatory Electrocardiogram | Nonlooping Event Recorder | Looping Event Recorder | External Cardiac Ambulatory Telemetry | Insertable Cardiac Monitor | |
---|---|---|---|---|---|---|
Cardiac intervals | First choice | Not recommended | Not recommended | Not recommended | Not recommended | Not recommended |
Heart rate range | Obtain as baseline | First choice | Second choice | Second choice | Second choice | Not recommended |
Daily dysrhythmia | Obtain as baseline | First choice | Second choice | Second choice | Second choice | Not recommended |
Weekly/monthly dysrhythmia, >2 minutes duration | Obtain as baseline | Second choice | First choice | Second choice | Second choice | Not recommended |
Weekly/Monthly dysrhythmia, <2 minutes duration | Obtain as baseline | Second choice | Not recommended | First choice | Second choice | Not recommended |
Syncope | Obtain as baseline | Second choice | Not recommended | First choice | First choice | Third choice |
For patients who experience palpitations during or after physical activity, a cardiac exercise test may be indicated. During the study, the patient’s ECG is constantly monitored and may provide useful clinical information. Lastly, an invasive cardiac electrophysiology study may be necessary to define the mechanism of a diagnosed cardiac dysrhythmia. In these studies, electrode catheters are placed within the heart to map the electrical impulses within the conduction system and myocardium. Programmed electrical stimulation is used to initiate arrhythmias to establish or confirm the diagnosis and locate the source of the abnormal rhythm. Once the mechanism is understood, catheter ablation techniques may be curative.10
|
Exercise testing has become an integral part of the evaluation of children with congenital and acquired cardiovascular disease. Children are seldom sitting or lying quietly during waking hours. The evaluation of the patient in the exercise physiology laboratory allows the clinician to assess the cardiovascular system in a state that is more likely to be reflective of the normal daily physical activities. Although most exercise laboratories in children’s hospitals were originally designed primarily for cardiac patients, their role is expanding and now includes testing of many noncardiac symptoms and diseases.
Optimal exercise performance requires a continuous meshing of multiple systems of organs.12,14 The roles of the heart and lungs are to provide adequate energy substrates to working skeletal muscle and to remove the end products of aerobic and anaerobic metabolism during exercise. Assuring proper exercise performance requires the seamless and continuous meshing of multiple systems of organs.13,14 Working together, the muscular, cardiovascular, and pulmonary systems must produce mechanical energy from chemical energy at the cellular level, with subsequent delivery and removal of substrates for energy production and by-products of muscle metabolism. All of this is accomplished while maintaining chemical and thermal homeostasis within narrow ranges.
At the cellular level, adenosine triphosphate is the chief source of chemical energy for the exercising muscle. The stores of adenosine triphosphate and phosphocreatine in the myocytes are sufficient only for 10 to 15 seconds of activity and thus must continuously be replenished anaerobically in the cytosol or aerobically in the mitochondria.13,15 Anaerobic activities use glucose, which is metabolized to pyruvate. Pyruvate is converted into lactic acid and excreted into the blood stream, where it is buffered by sodium bicarbonate converting it to lactate. This reaction results in the production of carbon dioxide and water, which are excreted in the lungs. The other fate of pyruvate, as well as fats, is aerobic metabolism in the Krebs cycle. Adenosine triphosphate is produced in large quantities via the electron transport chain, with oxygen functioning as the terminal electron acceptor, again producing carbon dioxide and water as by-products.14,15
During any activity, the availability and use of substrates will vary depending on the type, intensity, and duration of activity. The ratio of carbon dioxide production to oxygen consumption, abbreviated Vco2/Vo2, is called the respiratory exchange ratio (RER) or, if in a steady state, the respiratory quotient. In a state of high use of fat, the ratio is approximately 0.7. Conversely, during pure carbohydrate metabolism, the ratio is 1.0, reflecting the lower amount of oxygen needed to oxidize carbohydrates. The resting RER in the well-fed state will usually range from 0.8 to 0.9.
During a typical graded maximal exercise test, the work rate is gradually increased over the course of 10 to 15 minutes. Production of adenosine triphosphate will need to increase as mechanical work increases, and at low levels of work, this increase is met predominately by increased aerobic metabolism. As work rate increases, consumption of oxygen increases in a linear fashion (Figure 2-8). Near peak work rates, consumption will tend to plateau, as maximal Vo2 is achieved. This phenomenon is often absent in children.14,16,17
Figure 2-8

The relationship between consumption of oxygen Vo2 and rate of work during progressive exercise in a healthy and well-conditioned adolescent. Note that, with the onset of exercise, there is an essentially linear relationship between these 2 features. Close to the peak of exercise, the consumption of oxygen levels off, despite the continued rise in rate of work. (Reproduced with permission, from Stephens P Jr, McBride MG, Paridon SM. Cardiopulmonary Stress Testing. In: Paediatric Cardiology. 3rd ed. edited by Anderson RH et al. (Philadelphia, PA: Churchill Livingstone/Elsevier, 2010), 415-436.)
As Vo2 increases in response to increased work rate, there is a gradual rise in the RER with a gradual shift in use of carbohydrates as an energy source, allowing for more efficient use of oxygen. Second, the rise in the ratio occurs as a result of increased levels of lactate in the blood. At approximately 50% to 60% of the maximal Vo2, levels of lactate begin to rise in the serum. This point is known as the lactate threshold and is the onset of anaerobic metabolism by the exercising muscles. Above the threshold, levels of lactate rise exponentially as work rate increases, necessitating increased buffering by sodium bicarbonate in order to maintain blood pH homeostasis. The by-product of the buffering process, carbon dioxide, must be removed in order to maintain levels within the normal range. This causes a significant rise in production of carbon dioxide out of proportion to the rise in consumption of oxygen, resulting in the RER increasing to greater than 1.0. The RER in adults at peak exercise may be as high as 1.2 to 1.4 but is usually somewhat lower in children.16-21 The increase in production of carbon dioxide associated with the buffering of lactate allows for measurement of a noninvasive surrogate of the lactate threshold. This surrogate, known as the ventilatory anaerobic threshold, is defined as the point where production of carbon dioxide and minute ventilation begin to rise out of proportion to the consumption of oxygen (Figure 2-9). Unfortunately, the ventilatory anaerobic threshold can be difficult to measure accurately in smaller children who tend to have erratic patterns of breathing.16-21
Figure 2-9

A. The relationship of minute ventilation (VE) to rate of work in the same subject as in Figure 2-8. Note there is a steady rise in minute ventilation as rate of work increases. B. The ventilatory equivalents of oxygen (VE/Vo2) and carbon dioxide (VE/Vco2) for the same subject. The onset of the ventilatory anaerobic threshold (VAT) is marked. (Reproduced with permission, from Stephens P Jr, McBride MG, Paridon SM. Cardiopulmonary Stress Testing. In: Paediatric Cardiology. 3rd ed. edited by Anderson RH et al. (Philadelphia, PA: Churchill Livingstone/Elsevier, 2010), 415-436.)
The rise in Vo2 during exercise is dependent on increases in stroke volume, heart rate, and widening of the difference in the content of oxygen in arterial and mixed venous blood. During strenuous exercise, cardiac output may rise as much as 5-fold over resting levels, with both stroke volume and heart rate contributing to this increase15,21-25 (Figure 2-10). By a combination of autonomic and metabolic vasoregulatory mechanisms, blood flow is preferentially shunted to the exercising muscles (Figure 2-11). At peak exercise, blood flow to exercising muscle may be 80% or more of the total cardiac output.26-28 Because of the difficulties in measuring cardiac output during exercise and the linear relationship of Vo2 with cardiac output over a wide range, it is the most commonly used surrogate of cardiac output during exercise testing.14,26,29,30
Figure 2-10

The relationship of heart rate and stroke volume to increasing consumption of oxygen during cycle ergometry in 23 male and female subjects. Note that stroke volume reaches its maximal value at approximately 30% to 40% of the maximal uptake of oxygen. Heart rate continues to rise in a linear fashion throughout exercise. (Reprinted, with permission, from Astrand P, Rodahl K. The Muscle and Its Contraction. Textbook of Work Physiology, Physiological Bases of Exercise. 3rd ed., Vol. 2. New York, NY: McGraw-Hill, Inc.; 1986:12-53.)
Figure 2-11

The parallel circuits of flow through the various systems of organs, both at rest and during peak exercise. Note that the cardiac output increases by approximately 5-fold from rest to strenuous exercise. The relative distribution of flow to the various systems, in contrast, is significantly different from rest to peak exercise. In both states, the red squares are proportional to the percentage of cardiac output received by the particular system. Note that the flow of blood to the muscle increases from approximately 15% to 20% of cardiac output at rest to 80% to 85% of the cardiac output at peak exercise. (Reprinted, with permission, from Astrand P, Rodahl K. The Muscle and Its Contraction. Textbook of Work Physiology, Physiological Bases of Exercise. 3rd ed., Vol. 2. New York, NY: McGraw-Hill, Inc.; 1986:12-53.)
Consumption of oxygen and removal of carbon dioxide require that the cardiovascular and pulmonary systems work together as a single integrated unit. For this reason, there is a very tight relationship between minute ventilation (VE), Vo2, and Vco2. Such a relationship is demonstrated in ventilatory equivalents for carbon dioxide and oxygen, namely VE/Vco2 and VE/Vo2. The typical relationship of minute ventilation to increasing work rate and the relationship of VE/Vco2 and VE/Vo2 to work rate are depicted in Figure 2-9.14,18-20
Adequate space and environmental controls are important in order to ensure a successful exercise test. Sufficient space is needed to accommodate the various ergometers and monitoring equipment, including emergency resuscitation equipment, while maintaining adequate space to access the patient in emergency situations.31 The climate of the laboratory must be well controlled for temperature and humidity to allow proper thermoregulation during the exercise test.32
Exercise testing has been performed in children with very low risk, even in those who have complex disease.33,34 Although significant complications of exercise testing are rare, proper safety precautions are essential. Key staff usually include at least one physician who is well trained in pediatric exercise testing. A physician does not need to be present for testing patients deemed to be at low risk or healthy32,35 but should be present at the testing of any child deemed to be at increased risk of a complication, such as a child with a life-threatening arrhythmia or syncope. The American Heart Association published guidelines for patients who are at low risk for exercise complications that require a physician to be available, but not physically present (Table 2-2).35 The risk for each patient must be individually assessed.
|
In most pediatric exercise laboratories, testing is primarily directed toward measuring aerobic capacity. Therefore, ergometers should generate work in the large muscle groups. The 2 types of ergometers most commonly used are the motorized treadmill and the upright cycle ergometer. The choice of ergometer depends on the type of information desired, but there are both advantages and disadvantages to each modality (Table 2-3).36-38 Therefore, it is best that a laboratory be equipped to perform testing using either type of ergometer.
Features | Treadmill | Cycle |
---|---|---|
Patient familiarity | + | |
Higher work rates and oxygen consumption | + | |
Greater pediatric experience | + | |
Quantification of work performed | + | |
ECG and blood pressure artifact | + | |
Safety | + | |
Expense | + | |
Noise | + |
Many high-quality commercial ECG recording systems are currently available. No system currently available is designed specifically for pediatric use, but all are generally acceptable without modification. Minimization of distortion of signals is accomplished with computer digitization of the analog electrical signal. The printer should use a direct writer mechanism.36
The use of these analyzers has become routine in laboratories studying pediatric exercise physiology. Measurements of consumption of oxygen, production of carbon dioxide, and pulmonary functions such as minute ventilation, tidal volume, and respiratory rate are easily obtainable. Several systems at reasonable prices are commercially available. In addition to measuring expired gases, these systems are frequently equipped to perform resting spirometry and to receive input from other sources, such as an ECG recorder. This allows a single system to generate complete and final reports.
The Bruce protocol and its modifications are the most commonly used treadmill protocols in the pediatric exercise laboratory, consisting of 3-minute stages with an increase in both speed and grade of the treadmill at each stage.38 In the last decade, protocols with shorter stages, usually of 1 or 2 minutes, and smaller incremental increases in rates of work have gained popularity. These protocols, such as the Balke treadmill protocol, may use a fixed speed, with increases only in grade, or may increase both speed and grade.
The most commonly used cycle protocol is that devised by James, which consists of 10 stages, each lasting 3 minutes.39 The incremental increases for each stage vary depending on body surface area. Collection of expired gas is such a common occurrence that many laboratories are now using ramp cycle protocols. In this protocol, the rate of work is increased in small, frequently single-watt increments, thus producing a smooth continuous rise throughout the test.31,37,38 Protocols are shown in Figures 2-12 and 2-13.
Figure 2-12

The panels show the protocols for testing using a treadmill. A. The Bruce protocol consists of 3-minute stages with an increase in both speed and grade. The first 2 stages are often omitted in healthy adult testing. B. In the Balke protocol, the grade is increased from 0% to 2% after the first minute and increased 1% in each subsequent minute. The speed of the treadmill is held constant at 3.5 mph. (Reproduced with permission, from Stephens P Jr, McBride MG, Paridon SM. Cardiopulmonary Stress Testing. In: Paediatric Cardiology. 3rd ed. edited by Anderson RH et al. (Philadelphia, PA: Churchill Livingstone/Elsevier, 2010), 415-436.)
Figure 2-13

Protocols for use with an upright cycle ergometer. A. The James protocol. The initial rate of work is 200 kpm/min. The rate is increased every 3 minutes by different amounts, depending on the body surface area (BSA) of the patient. B. The ramp protocol. The patient initially pedals for 3 minutes with unloaded cycling to establish a baseline metabolic state. The rate of work is then increased continuously at a chosen level based on the physical condition, age, and size of the patient. (Reproduced with permission, from Stephens P Jr, McBride MG, Paridon SM. Cardiopulmonary Stress Testing. In: Paediatric Cardiology. 3rd ed. edited by Anderson RH et al. (Philadelphia, PA: Churchill Livingstone/Elsevier, 2010), 415-436.)
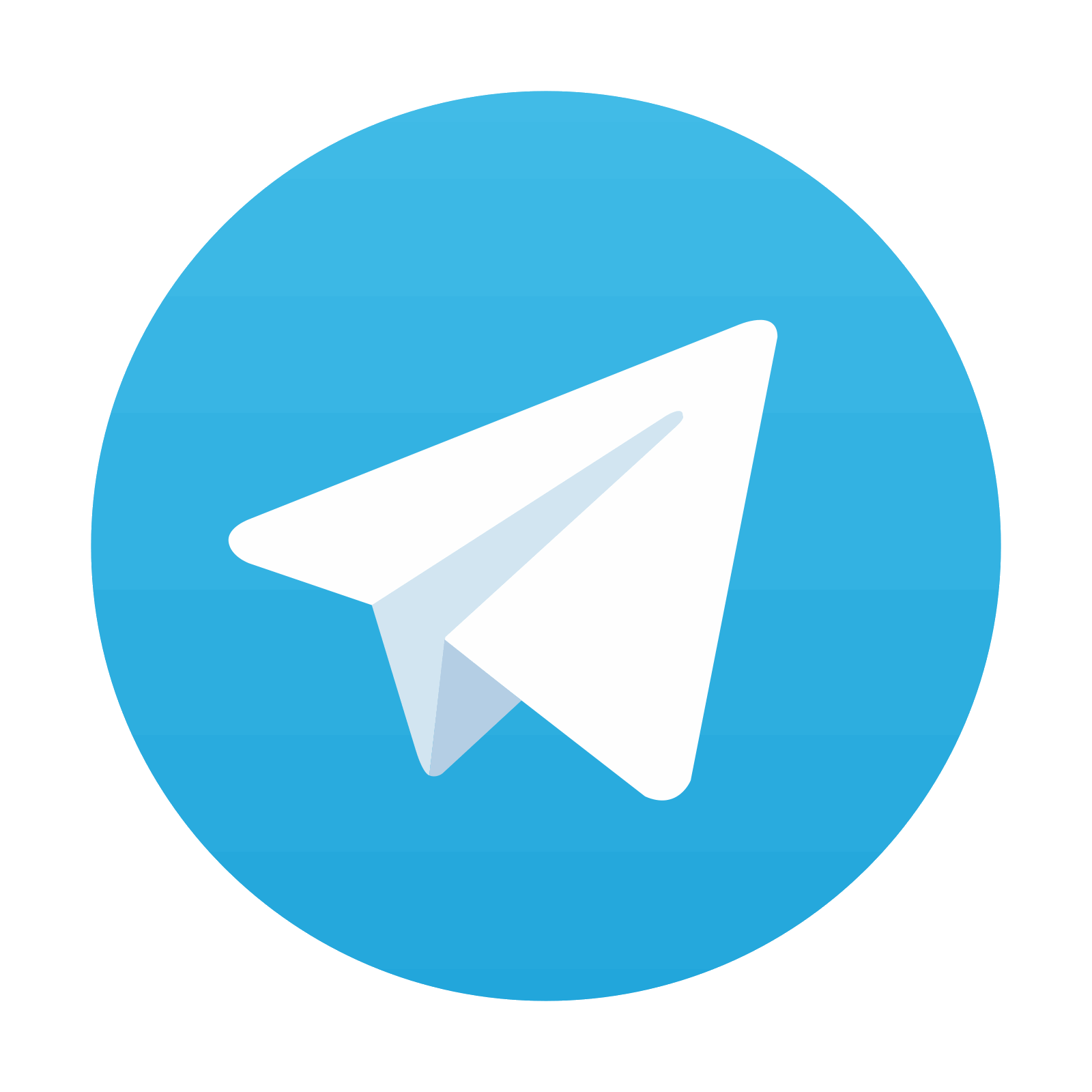
Stay updated, free articles. Join our Telegram channel
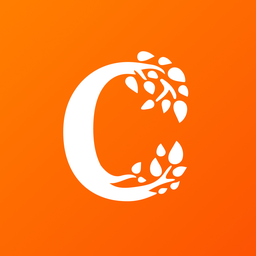
Full access? Get Clinical Tree
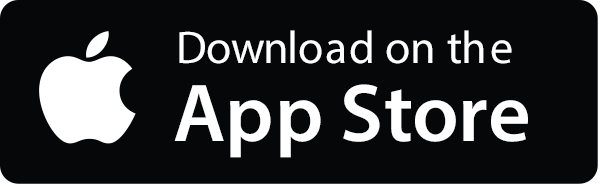
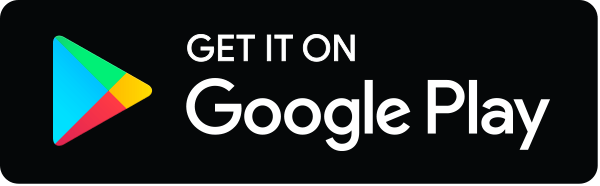
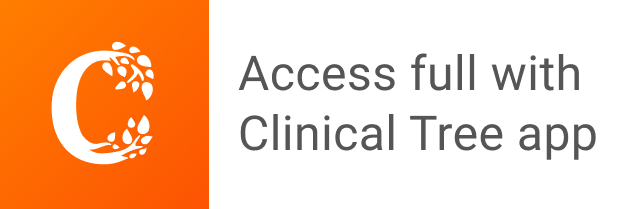