A cardiac surgical procedure is the most acute application of basic dynamic physiology that exists in medical care. Basic physiologic concepts of electromechanical activation and association, loading conditions, inotropy, etc all affect a successful outcome. Working knowledge of these fundamental concepts is imperative to maintain and return a patient to normal function. The purpose of this chapter is to present a manageable outline of cardiac physiology that can be used in daily practice, as a framework against which pathologic processes can be measured, assessed, and treated.
The heart beats continuously based on the unique features of its component cells. A cardiac cycle begins when spontaneous depolarization of a pacemaker cell initiates an action potential. This electrical activity is transmitted to atrial muscle cells and to the conduction system which transmits the electrical activity to the ventricle. Activation is dependent on components of the cell membrane and cell which induce and maintain the ion currents that promote and spread electrical activation.
The activity of cells in the heart is triggered by an action potential. An action potential is a cyclical activation of the cell comprised of a rapid change in the membrane potential (the electrical gradient across the cell membrane) and subsequent return to a resting membrane potential. This process is dependent on a selectively permeable cell membrane and proteins that actively and passively direct ion passage across the cell membrane. The specific components of the myocyte action potential are detailed in Figure 3-3. The myocyte action potential is characterized by a rapid initial depolarization mediated by fast channels (sodium channels), then a plateau phase mediated by slow channels (calcium channels). Further details of this process are introduced as their components are described.
The cardiac cell is surrounded by a membrane (plasmalemma or more specific to a muscle cell, sarcolemma). The structural components of the sarcolemma allow for the origination and then the conduction of an electrical signal through the heart with subsequent initiation of the excitation-contraction coupling process. The sarcolemma also participates in the regulation of excitation, contraction, and intracellular metabolism in response to neuronal and chemical stimulation.
The sarcolemma is a phospholipid bilayer that provides a barrier between the extracellular compartment and the intracellular compartment or cytosol. The sarcolemma, which is only two molecules thick, consists of phospholipids and cholesterol aligned so that the lipid, or the hydrophobic, portion of the molecule is on the inside of the membrane, and the hydrophilic portion of the molecule is on the outside (Figure 3-1). The phospholipid bilayer provides a fluid barrier that is particularly impermeable to the diffusion of ions. Small lipid-soluble molecules such as oxygen and carbon dioxide diffuse easily through the membrane. The water molecule, although insoluble in the membrane, is small enough that it diffuses easily through the membrane (or through pores in the membrane). Other, slightly larger molecules (sodium, chloride, potassium, calcium) cannot easily diffuse through the lipid bilayer and require specialized channels for transport.1,2
Figure 3-1
The sarcolemma is a bilayer in which phospholipid and cholesterol molecules are arranged with hydrophobic domains within the membrane and hydrophilic domains facing outward. The membrane-spanning protein shown here is similar to many ion channels, with six hydrophobic alpha-helices spanning the membrane and surrounding a central channel.

The specialized ion-transport systems within the sarcolemma consist of membrane-spanning proteins that float in and penetrate through the lipid bilayer. These proteins are associated with three different types of ion transport: (1) diffusion through transmembrane channels that can be opened or closed (gated) in response to electrical (voltage-gated) or chemical (ligand-gated) stimuli; (2) exchange of one ion for another by attachment to binding sites for transmission in response to an electrochemical gradient; and (3) active (energy-dependent) transport of ions against an electrochemical gradient.
Other proteins located in the sarcolemma serve as receptors for neuronal or chemical control of cellular processes.
Most of the voltage-gated channels consist of four subunits that surround the water-filled pore through which ions cross the membrane. A schematic diagram of an ion channel is shown in Figure 3-2. Each channel contains a selectivity filter that allows the passage of particular ions based upon pore size and electrical charge, and an activation gate regulated by conformational changes induced by either a voltage-sensitive or a ligand-binding region of the protein. Many channels also have an inactivation gate.1,3
Figure 3-2
A voltage-gated sodium channel is schematically depicted. The shaded region is the selectivity filter. A represents the activation gate, and I represents the inactivation gate. At rest, the inactivation gate is open and the activation gate is closed. As the transmembrane potential rises from –80 to –60 mV, the activation gate opens, and sodium ions pass through the channel. Within a few milliseconds, the inactivation gate closes. Once the cell repolarizes the resting ion channel returns to the resting state.

The voltage-gated sodium channel is prominent in most electrically excitable muscle and nerve cells. Energy-dependent pumps and other ions create a large concentration gradient of positive sodium ions (142 mEq/L outside, 10 mEq/L inside) and a large electrical gradient (–70 to –90 millivolts (mV) from outside to inside) across the cell membrane. Both gradients favor the influx of sodium. This passive influx is termed an inward current. The inward current of sodium ions begins to depolarize (reduce the electrical gradient) across the sarcolemma. When the membrane potential is raised to between –70 and –50 mV, the activation gate of the sodium channel opens. Sodium ions rapidly rush into the cell depolarizing the sarcolemmal membrane. The inactivation gate of the sodium channel begins to close at the same voltage, with a built-in time delay, so the sodium channel is open for only a few milliseconds. Because these channels open and close so quickly, they are called fast channels. The inactivation gate of the sodium channel remains closed until the cell is repolarized to the resting negative membrane potential.4,5
There are two important calcium channels. The type T (transient)-calcium channels open as the membrane potential rises to –60 to –50 mV, and then close quickly. These T-calcium channels are important in early depolarization, especially in atrial pacemaker cells.
The second major calcium channel, type L (long-lasting) channel, a slow channel, leads to an inward (depolarizing) current that is slowly inactivated and therefore prolonged. These channels open at a less negative potential (–30 to –20 mV). Once open, the prolonged inward calcium current (Figure 3-3) sustains the action potential. This increase in cytosolic calcium begins the excitation-contraction sequence. Beta-receptor stimulation induces conformational changes in the channel, resulting in an increased influx of calcium ions and an associated increase in the strength of sarcomere contraction. This effect is attenuated by stimulation of acetylcholine and adenosine receptors.6,7
Figure 3-3
A typical ventricular myocyte action potential and the ion currents contributing to it are schematically represented. Inward (depolarizing) currents are depicted as positive, and outward (repolarizing) currents are depicted as negative. The horizontal filled bars show the state of the gate of the ion channel (white = open; black = closed; shaded = partially open). In the case of the sodium channel, both the activation and inactivation gates are shown (Ca = calcium; i = current; K = potassium; Na = sodium).

A variety of potassium channels, both voltage- and ligand-gated, are present in cardiac cells. Three voltage-gated potassium channels moderate the delayed rectifier current which repolarizes the cell membrane (Figure 3-3).8
Several ligand-gated potassium channels have been identified. Acetylcholine and adenosine-activated potassium channels are time-independent, and lead to hyperpolarization in pacemaker and nodal cells, thereby delaying spontaneous depolarization. A calcium-activated potassium channel opens in the presence of high levels of cytosolic calcium and probably enhances the delayed rectifier current, leading to early termination of the action potential. An adenosine triphosphate (ATP)-sensitive potassium channel is closed in the metabolically normal myocyte, but is opened in the metabolically starved myocyte in which ATP stores have been depleted, leading to hyperpolarization of the cell, thereby retarding depolarization and contraction.
The sodium-potassium pump uses the energy obtained from the hydrolysis of ATP to move three Na+ ions out of the cell and two K+ ions into the cell, each against its respective concentration gradient. Since there is a net outward current (three Na+ ions for two K+ ions), the pump contributes about 10 mV to the resting membrane potential. The activity of the pump is strongly stimulated by attachment of sodium to its binding site. The Na+-K+ adenosine triphosphatase, Na+-K+ (ATPase), has a very high affinity for ATP, so that the pump continues to function even if ATP levels are reduced.
The ATP-dependent calcium pump transports calcium out of the cell against a strong concentration gradient. This action represents a net outward current, but the magnitude of this current is quite small because the bulk of calcium transferred out of the cell occurs with sodium-calcium exchange (described in the following). The cytosolic protein, calmodulin, can complex with calcium and facilitate the action of the pump; thus, increased intracellular calcium levels stimulate the pump.9,10
Multiple proteins that traverse the membrane allow ion exchanges using the potential energy of the electrochemical gradient which favors the influx of sodium. The sodium-calcium exchanger exchanges three extracellular sodium ions for one intracellular calcium ion, leading to a net single positive charge transported into the cell with each exchange. The exchange system is sensitive to the concentration of sodium and calcium on both sides of the membrane, and to the membrane potential. If external sodium concentrations decrease, the driving force for removal of calcium from the cell is decreased, leading to an increase in cytosolic calcium (and a consequent increase in contraction). Thus, hyponatremia can increase cardiac contractility. If the intracellular sodium concentration increases, as occurs with ischemia, the gradient for sodium influx is reduced, and the pump slows down or actually reverses, extruding sodium in exchange for an influx of calcium. This mechanism may be central to the accumulation of calcium during ischemia. The sodium-calcium exchange mechanism has a maximum exchange rate that is some 30 times higher than the sarcolemmal ATP-dependent calcium pump and is the primary mechanism for removal of excess cytosolic calcium.7
The sodium-hydrogen exchanger extrudes one intracellular hydrogen ion in exchange for one extracellular sodium ion, and is electrically neutral. This pump prevents intracellular acidification. Acidification (eg, during ischemia) increases the affinity of the pump for H+ ions, promoting the removal of H+ preserving intracellular pH at the expense of sodium accumulation. The accumulation of sodium ions may then trigger reversal of the sodium-calcium exchange pump to favor the accumulation of calcium within the cell. This is a possible mechanism underlying injury or cell death during ischemia-reperfusion.
To allow concurrent activation of all the myofibrils in the muscle cell, the electrical activation signal must be rapidly and evenly spread through all portions of the cell. This is accomplished through the t-tubules, and the subsarcolemmal cisternae and sarcotubular network of the sarcoplasmic reticulum.
The basic contractile unit in a muscle cell is the sarcomere. Sarcomeres are joined together in the myofibril at the z-lines. A system of transverse tubules (t-tubules) extends the sarcolemma into the interior of the cardiac cell (Figure 3-4). These tubules are perpendicular to the sarcomere, near the z-lines, extending the extracellular space close to the contractile proteins. The transverse tubules contain the calcium channels, which are in close relationship to the foot proteins of the subsarcolemmal cisternae.
The sarcoplasmic reticulum is a membrane network within the cytoplasm of the cell surrounding the myofibrils. The primary function of the sarcoplasmic reticulum is excitation-contraction coupling by sudden release of calcium to stimulate the contraction proteins and then rapid removal of this calcium to allow relaxation of the contractile elements. The subsarcolemmal cisternae and the sarcotubular network are the two portions of the sarcoplasmic reticulum that mediate this process.
The subsarcolemmal cisternae are near the sarcolemma and the t-tubules. Foot proteins are found in the membrane of the sarcoplasmic reticulum, with a large protein component extending into the gap between the subsarcolemmal cisternae and the sarcolemma of the t-tubule. The foot proteins respond to the release of calcium by opening a calcium channel, which allows the release of a much larger quantity of calcium from the subsarcolemmal cisternae. This is “calcium-triggered” calcium release with calcium transported across the sarcolemma, leading to calcium release from the subsarcolemmal cisternae. The magnitude of calcium release from the subsarcolemmal cisternae appears to be related to the magnitude of the trigger. The calcium channels then close and the calcium is returned to the sarcoplasmic reticulum by an ATP-dependent calcium pump located in the sarcotubular network.1,10 The sarcotubular network is the portion of the sarcoplasmic reticulum that surrounds the contractile elements of the sarcomere (Figure 3-5).
Figure 3-5
Anatomy of the cardiac sarcomere. Ca2+ influx during excitation provokes the release of additional Ca2+ from the sarcoplasmic reticulum (SR). The Ca2+ binds to troponin on the thin filaments triggering sarcomeric contraction (systole). The Ca2+ is then removed from the cytosol through uptake into the SR and extracellular extrusion allowing sarcomere relaxation (diastole). (Reproduced with permission from Kobirumaki-Shimozawa F, Inoue T, Shintani SA, et al: Cardiac thin filament regulation and the Frank-Starling mechanism, J Physiol Sci. 2014 Jul;64(4):221-232.)

Regulation of calcium transport by the cardiac sarcoplasmic reticulum occurs primarily at the site of the calcium pump. A calcium-calmodulin complex phosphorylates the pump to stimulate pump activity. Reduced ATP availability will slow pump function. Phospholamban inhibits the basal rate of calcium transport by the calcium pump. This inhibition is reversed when phospholamban is phosphorylated by a cyclic AMP-or calcium-calmodulin–dependent protein kinase. This is a very important mechanism for beta-adrenergic regulation; cyclic AMP levels increase with activation of the beta-adrenergic receptor. As phospholamban is phosphorylated, there is accelerated calcium turnover and increased sensitivity of the calcium pump, which facilitates uptake of calcium from the cytosol and relaxation of the heart. Phosphorylation of phospholamban does not affect the sarcolemmal calcium pump, thereby tending to favor retention of calcium within the cell (increasing the calcium content of the sarcoplasmic reticulum at the expense of calcium removed from the cell through the sarcolemma). This might lead to an increased pulse of calcium within the cell, thereby favoring increased contractility.7,10 Phosphorylation of phospholamban, in the presence of intracellular calcium, stimulates calcium uptake to protect the heart from calcium overload.
In this ionic milieu, the importance of intracellular pH maintenance should be stressed. Regulation of intracellular pH is complex and beyond the scope of this text, but a few simple principles are important to review. Reduced intracellular pH diminishes the amount of calcium released from the sarcoplasmic reticulum and reduces the responsiveness of myofilaments to calcium. Elevation of the pH will have the opposite effect. The clinical relevance of this observation cannot be overstressed.
The state of the cardiac cell is determined by a balance of forces based on electrical and chemical gradients. At rest (during diastole), the cardiac cell is polarized. The electrical potential across the sarcolemma is primarily determined by the concentration gradient of potassium across the membrane. This gradient is established by the sodium-potassium pump. However, once this pump shuts off, the steady state is determined by the balance of electrical and chemical forces. The sarcolemma is impermeable to some ions, permeable to others, and selectively permeable to others. Steady-state properties of a mixture of ions of variable permeabilities across a membrane are described by the Gibbs-Donnan equilibrium.11 The sarcolemma prevents the diffusion of large anions (eg, proteins and organic phosphates). At rest, the sarcolemma is relatively permeable to potassium ions because of the open state of most potassium channels, but less permeable to sodium. The concentration gradient established by the sodium-potassium pump promotes the efflux of potassium ions across the sarcolemma. The outward flow of positive ions is counterbalanced by the increasing electronegativity of the interior of the cell owing to the impermeant anions. A Gibbs-Donnan equilibrium is established such that the electronegativity of the cell interior retards potassium-ion efflux to the same degree that the concentration gradient favors K+ efflux. At equilibrium, the forces balance with an intracellular potassium concentration of 135 mM and extra-cellular concentration of 4 mM and a predicted resting membrane potential of –94 mV. The actual resting membrane potential is measured at about –90 mV because of smaller contributions from the current of other less permeable ions (eg, sodium and calcium). However, the potassium current is the main determinant of the resting membrane potential.12
The action potential represents the triggered response to a stimulus derived either internally (slow depolarizing ionic currents) or externally (depolarization of adjacent cells). A typical fast-response action potential which occurs in atrial and ventricular myocytes and special conduction fibers is depicted in Fig. 3-3. As the transmembrane potential decreases to approximately –65 mV, the “fast” sodium channels open. These channels remain open for a few milliseconds when the inactivation gate of the “fast” sodium channel closes. The large gradient of sodium ions promotes rapid influx, depolarizing the cell to a slightly positive transmembrane potential: phase 0 of the action potential. A transient potassium current (ito) causes a very early repolarization (phase 1) of the action potential, but this fast channel closes quickly. The plateau of the action potential (phase 2) is sustained at a neutral or slightly positive level by an inward flowing calcium current, first from the transient calcium channel and second through the long-lasting calcium channel. The plateau is also sustained by a decrease in the outward potassium current (iK1). With time, the long-lasting calcium channels begin to close, and the repolarizing potassium current (iK, the delayed rectifier current) leads to the initiation of phase 3 of the action potential. As repolarization progresses, the stronger first potassium current (iK1) dominates, leading to full repolarization of the membrane to the resting negative potential. During the bulk of the depolarized interval (phase 4) the first potassium current predominates in myocytes.
The sodium channels cannot respond to a second wave of depolarization until the inactivation gates are reopened (by repolarization during phase 3). As a result, the membrane is refractory to the propagation of a second impulse during this time interval, referred to as the absolute refractory period. As the membrane is repolarized during early phase 3 of the action potential, and some of the sodium channels have been reactivated, a short interval exists during which only very strong impulses can activate the cell, which is termed the relative refractory period. A drug that acts to speed up the inactivation gate will shorten both the absolute and the relative refractory periods.1,13,14
The action potential of the slow response cells of the nodal tissue (sinoatrial node, or SA node, and atrioventricular node, or AV node) differs from that in the fast-response cells, as shown in Figure 3-6. The rapid upstroke of phase 0 is less prominent due to the absence of fast Na+ channels. Phase 1 is absent, as there is no rapid inward potassium current. In addition, the plateau phase (phase 2) is abbreviated because of the lack of a sustained active Na+ inward current, and the lack of sustained calcium current. The repolarization phase (phase 3) leads to a resting phase (phase 4) that begins to depolarize again, as opposed to the relatively stable resting membrane potential of myocytes. The slowly depolarizing phase 4 resting potential is called the diastolic depolarization current, or the pacemaker potential. Continued depolarization of the membrane potential ultimately reduces it to the threshold potential that stimulates another action potential. This diastolic depolarization potential is the mechanism of automaticity in cardiac pacemaker cells. Diastolic depolarization is caused by the concerted and net actions of: (1) a decrease in the outward K+ current during early diastole (phase 4); (2) persistence of the slow inward Ca2+ current; and (3) an increasing inward Na+ current during diastole. The inward Na+ current most likely predominates in nodal and conduction tissue. The slope of the diastolic depolarization determines the rate of action-potential generation in the pacemaker cells, and is the primary mechanism determining heart rate. Of all the cardiac cells, the fastest rate of depolarization is in the SA node, and action potentials are generated at a rate of 70 to 80 per minute. The AV node is a slower rate of depolarization, at 40 to 60 times per minute. The ventricular myocytes are the slowest, at 30 to 40 times per minute. Once a depolarization is initiated in a pacemaker cell and propagated, it will depolarize the remainder of the heart in a synchronized and sequential manner. The heart rate can be altered by changing the slope of the diastolic depolarization (eg, acetylcholine decreases the slope and heart rate; beta-adrenergic agonists increase the slope and heart rate). If the slope is unchanged, hyperpolarization (more negative resting potential) or raising the threshold potential will increase the time to reach threshold, thus decreasing the heart rate.
Figure 3-6
The membrane potential of a spontaneously depolarizing cell of the sinoatrial node, and the ion currents contributing to it. Inward (depolarizing) currents are depicted as positive, and outward (repolarizing) currents are depicted as negative (Ca = calcium; i = current; K = potassium; Na = sodium).

Each myocyte is mechanically anchored and electrically connected to the next myocyte by an intercalated disc at the end of the cell. These discs contain gap junctions that facilitate flow of charged molecules from one cell to the next. These pores are composed of a protein, connexin. Permeability through the cardiac gap junction is increased by both ATP- and cyclic AMP-dependent kinases. This allows the gap junctions to close if ATP levels fall, thereby reducing electrical and presumably mechanical activity, which is essential in limiting cell death when one region of the heart is damaged. It also allows conduction to increase when cyclic AMP increases in response to adrenergic stimulation.
After spontaneous depolarization occurs in the pacemaker cells of the SA node, the action potential is conducted throughout the heart. Special electrical pathways facilitate this conduction. Three internodal paths exist through the atrium between the SA node and the AV node. After traversing the AV node, the action potential is propagated rapidly through the bundle of His and into the Purkinje fibers located on the endocardium of the left and right ventricles. Rapid conduction through the atrium causes contraction of most of the atrial muscle synchronously (within 60 to 90 ms).Similarly, the rapid conduction of the signal throughout the ventricle leads to synchronous contraction of the bulk of the ventricular myocardium (within 60 ms). The delay in the propagation of the action potential through the AV node by 120 to 140 ms allows the atria to complete contraction before the ventricles contract. Slow conduction in the AV node is related to a relatively higher internal resistance because of a small number of gap junctions between cells, and slowly rising action potentials.
Normally the SA node spontaneously depolarizes first, such that the cardiac beat originates from this primary pacemaker site. If the SA node is damaged or slowed by vagal stimulation or drugs (eg, acetylcholine), ectopic pacemakers in the atrium, AV node, or the His-Purkinje system can take over. Occasionally, aberrant foci in the heart spontaneously depolarize, thereby leading to aberrant or “premature” contractions from the atrium or the ventricle. These contractions ordinarily do not interfere with the normal depolarization of the heart.
Reentry arrhythmias are perhaps the most common dangerous cardiac rhythm. Ordinarily, the action potential depolarizes the entire atrium or the entire ventricle in a short enough time interval so that all of the muscle is refractory to further stimulation at the same time. A reentry arrhythmia is caused by propagation of an action potential through the heart in a “circus” movement. For reentry to occur there must be a unidirectional block (transient or permanent) to action potential propagation. Additionally, the effective refractory period of the reentered region must be shorter that the propagation time around the loop.12 For example, if a portion of the previously depolarized myocardium has repolarized before the propagation of the action potential is completed throughout the atrium or ventricle, then that action potential can continue its propagation into this repolarized muscle. Such an event generally requires either dramatic slowing of conduction of the action potential, a long conduction pathway, or a shortened refractory period (Figure 3-7). All of these situations occur clinically. Ischemia slows the sodium-potassium pump, which decreases the resting membrane potential and slows propagation of the action potential. Hyperkalemia decreases the resting membrane potential, which increases excitability and inactivates the sodium-potassium pump, slowing propagation of the action potential. Progressive atrial dilation creates a long conduction pathway around the atrium. Adrenergic stimulation shortens the refractory period.
Figure 3-7
Three conditions predisposing to reentry or “circus” pathways for action potential propagation are shown. Muscle that is refractory to action potential propagation is shown as black. Normally, as the action potential travels through the atrium or ventricle, all the muscle is depolarized sufficiently that the action potential encounters no more nonrefractory muscle and stops (A). If there is slowed conduction speed or a long pathway (B), the action potential may find repolarized (nonrefractory) muscle and continue in a circular path. Similarly, a shortened refractory period (C) may lead to rapid repolarization and predispose to a reentry and continuation of the action potential.

A special type of reentry arrhythmia occurs in Wolff-Parkinson-White syndrome in which an accessory pathway electrically connects the atrium and the ventricle. This accessory pathway can complete a circular electrical pathway between the atrium and the ventricle. Conduction is unidirectional across the AV node and the accessory pathway creates a loop that has a propagation time that is greater than the AV node refractory period, resulting in supraventricular tachycardia. In an alternative situation, because the accessory pathway does not have the inherent delay and refractory period of the AV node, rapid atrial tachycardias can be conducted in a 1:1 manner across the accessory pathway, leading to ventricular rates as fast as 300 beats per minute.
Numerous types of receptors are involved in regulating cardiovascular function. They include G-protein (GTP-binding proteins) coupled receptors, enzyme-linked receptors, ion channel-linked receptors, and nuclear receptors. Other ligands, such as nitric oxide, bind directly to their intracellular target.15 G-protein–coupled receptors are the most important. Ligand binding activates the synthesis of intracellular second messengers, protein kinases, and voltage-gated potassium channels.16 The most important second messenger is cyclic AMP, which transmits the response to sympathetic stimulation. Cyclic AMP is produced from ATP by adenylyl cyclase and broken down to AMP by phosphodiesterases. Cyclic AMP production is promoted by sympathetic and inhibited by parasympathetic stimulation. Another second messenger, cyclic guanosine monophosphate (GMP) is similarly produced, in response to nitric oxide and natriuretic peptides, by guanylyl cyclase and broken down by phosphodiesterases and opposes the actions of cyclic AMP.17 These and other second messengers activate signaling enzymes within the cell such as protein kinases.
Sympathetic fibers originate from the fourth and fifth thoracic spinal cord regions. Parasympathetic innervation derives through the vagus nerve connecting to the SA and AV nodes, atria, and blood vessels. Stretch receptors located in the atria and ventricles provide feedback to the central nervous system. Atrial natriuretic peptide, (similar to B-type natriuretic peptide which is clinically measured) is secreted by atrial myocytes in response to stretch and promotes natriuresis, diuresis, and smooth muscle relaxation. Stretch receptors on the posterior and inferior ventricular wall can trigger parasympathetic stimulation and inhibit sympathetic activity, leading to bradycardia and conduction block (von Bezold-Jarisch reflex).18
The parasympathetic nervous system is particularly important in control of the SA node. Acetylcholine released by the nerve endings of the parasympathetic system stimulates muscarinic receptors in the heart. The activated receptors produce an intracellular stimulatory G-protein that opens acetylcholine gated potassium channels. An increased outward (repolarizing) flow of potassium leads to hyperpolarization of the SA node cells. Stimulation of the muscarinic receptors also inhibits the formation of cyclic AMP, inhibiting the opening of calcium channels. A decreased inward flow of calcium, combined with an increased outward flow of potassium, leads to slowing of the spontaneous diastolic depolarization of the SA node cells (Figure 3-6). A similar effect in the AV node leads to slowing of conduction through the AV node.1
Sympathetic or adrenergic receptors in the heart affect heart rate, contractility, conduction velocity, and automaticity; and in the peripheral vasculature they affect smooth muscle contraction and relaxation. Alpha-adrenergic receptors cause vasoconstriction. There are two types of beta-adrenergic receptors: the beta1-adrenergic receptors, which predominate in the heart, and the beta2-adrenergic receptors, which are present in blood vessels and promote relaxation. The number of beta receptors per unit area (receptor density) of the sarcolemma can be upregulated or downregulated in response to various stimuli. Receptor sensitivity can also change depending on ambient conditions and variable stimuli.19 Cardiopulmonary bypass and ischemia cause downregulation of cardiac beta receptors. Acidemia causes desensitization of beta receptors. This is important in the perioperative period when acidemia can reduce cardiac contractility, systemic vascular tone, and the response to inotropic agents.
The beta1-adrenergic receptor couples with adenylyl cyclase (Figure 3-8). When the receptor site is occupied by an adrenergic agonist, a stimulatory G-protein is formed, which combines with GTP. This activated G-protein–GTP complex then promotes the activity of adenylyl cyclase, leading to the formation of cyclic AMP from ATP. The G-protein–GTP complex and the cyclic AMP actively promote calcium channel opening. The increased tendency for calcium channels to open during beta1-receptor stimulation increases cytosolic calcium and leads to a number of physiologic effects: (1) A positive chronotropic (heart rate) effect whereby the heart rate, conduction, and contraction velocity increase and the action potential is shortened, leading to a shortening of systole; (2) a positive dromotropic (conduction velocity) effect of accelerated conduction through the AV node; and (3) a positive inotropic (contractility) effect. Increased activity of the sarcoplasmic reticulum calcium pump (more rapid calcium uptake) leads to more rapid relaxation, which facilitates ventricular filling; (4) a positive lusitropic (relaxation) effect.20
Figure 3-8
Adrenergic stimulation via the action of beta agonists on beta receptors leads to a cascade of events in the myocyte, some of which are shown here. Note that an increase in cyclic AMP causes the activation of two inhibitory pathways, retarding excessively sustained adrenergic stimulation (cyclic AMP = cyclic adenosine monophosphate; Gs = stimulatory G-protein; GTP = guanosine triphosphate; SR = sarcoplasmic reticulum).

Two negative feedback systems diminish the response to beta agonists when stimulation is repetitive or persistent (tachyphylaxis). Increased cyclic AMP leads to: (1) increased phosphorylation of beta receptors leading to downregulation; and (2) increased activity of phosphodiesterase, the enzyme that degrades cyclic AMP. Acidosis will inhibit many steps in the sympathetic activation cascade, impairing contractility.
The activity spectrum of adrenergic receptors forms the basis of many therapeutic interventions; perioperatively to support cardiac function, and chronically to reduce mortality from myocardial infarction and treat congestive heart failure. The selectivity of the agonists and antagonists allows adaptation for various clinical scenarios. Some examples are detailed in Table 3-1.
Drug | Alpha | Beta1 | Beta2 | Clinical Usage | |
---|---|---|---|---|---|
Agonists | Epinephrine | Y | Y | Y | Low cardiac output, hypotension |
Norepinephrine | Y | Y | Hypotension | ||
Phenylephrine | Y | Hypotension | |||
Dobutamine | Y | Low cardiac output | |||
Dopamine | Y | Y | Low cardiac output, hypotension | ||
Isoproterenol | Y | Y | Bradycardia, low cardiac output, pulmonary hypertension | ||
Antagonists (beta-blockers) | Metoprolol | Y | Tachycardia, hypertension, MI, angina | ||
Atenolol | Y | Tachycardia, hypertension, MI, angina | |||
Esmolol | Y | Tachycardia, hypertension, MI, angina | |||
Carvedilol | Y (alpha-1) | Y | Y | Congestive heart failure |
Reduction in inotropy, lusitropy, chronotropy, and dromotropy by beta blockade will reduce myocardial oxygen consumption contributing to many of its beneficial effects. As beta blockade will lead to upregulation of sarcolemmal receptors, sudden cessation of beta blockade may cause a temporarily enhanced (and potentially dangerous) sensitivity to adrenergic stimulation.
Cyclic AMP plays a central role in the regulation of the cardiac cell. Cytosolic levels of cyclic AMP are also increased by activation of receptors other than beta receptors (ie, for histamine, dopamine, glucagon), and are decreased by inhibitory G-proteins produced by stimulation of muscarinic receptors by acetylcholine and by stimulation of adenosine receptors. Referring to Figure 3-8, one negative feedback response to the increase in cyclic AMP is an increase in phosphodiesterase, which breaks down cyclic AMP. Phosphodiesterase inhibitors (amrinone, milrinone) inhibit the breakdown of cyclic AMP and thereby increase its level in the cytosol. Their effect is synergistic to that of beta agonists. Because they do not stimulate the production of the G-protein–GTP complex, they have a lesser effect on calcium channel activation, and therefore less of the troublesome positive chronotropic and dromotropic effects of beta-adrenergic stimulation.21
There are four types of adenosine receptors. Adenosine receptors are linked to inhibitory and stimulatory G-proteins and various kinases. Activation of adenosine A1 receptors leads to inhibition of cyclic AMP production, inhibition of the slow calcium channel, and opening of an adenosine-activated ATP-sensitive potassium (KATP) channel. This leads to hyperpolarization, which delays conduction through the AV node and slows the ventricular response to atrial tachycardia.1,22 Pretreatment with adenosine confers a cardioprotective effect during ischemia and can inhibit the inflammatory responses initiated by ischemia and reperfusion.22
Angiotensin II is a vasoconstrictor and reduces renal fluid excretion. It is the final effector of the renin-angiotensin-aldosterone system. Renin, secreted by the juxtaglomerular apparatus in the kidney, splits angiotensin I from angiotensinogen, produced by the liver. Angiotensin I is converted to angiotensin II by the angiotensin-converting enzyme (ACE or kininase II, the target of ACE inhibitors) mainly in the lungs. Angiotensin II acts by causing: (1) vasoconstriction to increase systemic vascular resistance; and (2) stimulation of the adrenal cortex to secrete aldosterone, which increases fluid volume and thus cardiac output. Through both actions, angiotensin II modifies blood pressure. Angiotensin II receptor blockers (ARBs) directly inhibit angiotensin II subtype IA receptors.
The endothelins (ETs) have multiple effects. When bound to ET-A receptors they cause vasoconstriction, increased contractility, and proliferation. When bound to ET-B receptors they stimulate the release of nitric oxide and prostacyclin and have a vasodilatory effect.23
Bradykinins, acting through their receptors, cause vasodilation. Arginine vasopressin promotes reabsorption of water by the kidney and has a vasoconstrictor effect. Natriuretic peptides, released in response to atrial distension, promote diuresis and arteriolar dilatation.
Nitric oxide (NO) plays an essential role in cardiac excitation-contraction coupling. NO can regulate Ca2+ entry into the cardiomyocyte, and the release of NO in specific subcellular compartments can influence Ca2+ release from the sarcoplasmic reticulum. NO influences the contractile kinetics of the myofilaments, ensuring that Ca2+ homeostasis is closely matched with the activity of the contractile machinery and allowing for dynamic adjustments during the systolic and diastolic phase. Through the stimulus of cyclic-GMP production, low amounts of NO can increase contractility but higher doses attenuate cardiomyocyte contraction.17,24,25 Changes in cardiac NO production by nitric oxide synthase impact responses to heart failure, diabetes, atrial fibrillation and ischemia reperfusion states.26 Inhaled NO administered at low concentrations causes pulmonary arterial dilatation, reduces chronotropy, and has a positive inotropic effect.27
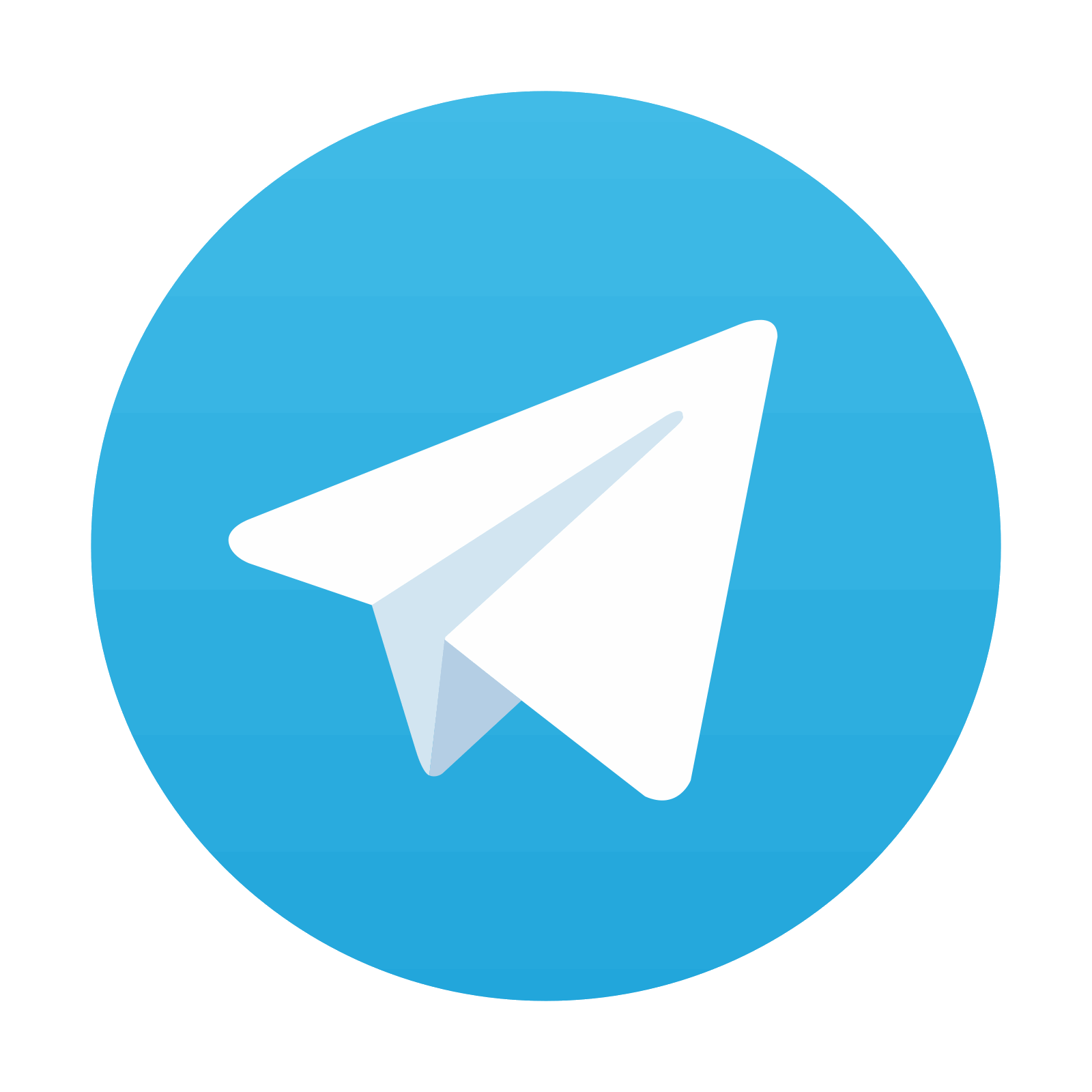
Stay updated, free articles. Join our Telegram channel
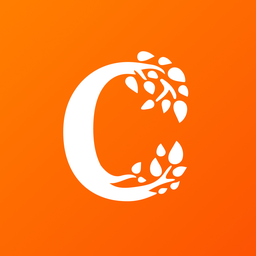
Full access? Get Clinical Tree
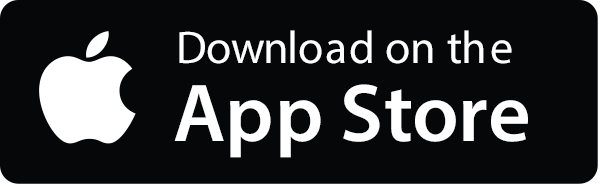
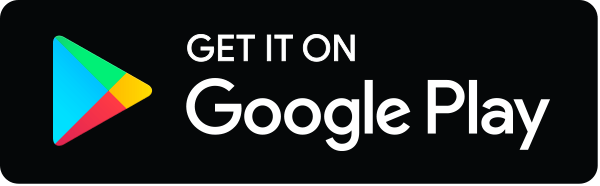