Clinical pharmacology associated with cardiac surgery is an important part of patient management. Patients in the perioperative period receive multiple therapeutic agents that affect cardiovascular and pulmonary functions. This chapter summarizes the pharmacology of the agents commonly used for treating the primary physiologic disturbances associated with cardiac surgery, hemodynamic instability, respiratory insufficiency, and alterations of hemostasis. For cardiovascular drugs, the common theme is that pharmacologic effects are produced by intracellular ion fluxes.
Several basic subcellular/molecular pathways are important in cardiovascular pharmacology, as shown in Fig. 4-1. The action potential in myocardial cells is a reflection of ion fluxes across the cell membrane, especially Na+, K+, and Ca2+.1,2 Numerous drugs used to control heart rate and rhythm act by altering Na+ (eg, lidocaine and procainamide), K+ (eg, amiodarone, ibutilide, and sotalol), or Ca2+ (eg, diltiazem) currents. Calcium also has a dominant effect on the inotropic state but by highly specialized intracellular mechanisms.3,4
FIGURE 4-1
Cardiac ion fluxes and the action potential. The resting membrane potential is largely a reflection of the intercellular/intracellular potassium gradient. Depolarization of the membrane during phase 4 triggers an initial fast sodium channel with overshoot (phase 0) followed by recovery (phase 1) to a plateau (phase 2) maintained by an inward calcium flux and then repolarization owing to an outward potassium flux (phase 3).

Myocardial contractility is a manifestation of the interaction of actin and myosin, with conversion of chemical energy from adenosine triphosphate (ATP) hydrolysis into mechanical energy. The interaction of actin and myosin in myocytes is inhibited by the associated protein tropomyosin. This inhibition is “disinhibited” by intracellular calcium. A similar situation occurs in vascular smooth muscle, where the interaction of actin and myosin (leading to vasoconstriction) is modulated by the protein calmodulin, which requires calcium as a cofactor. Thus intracellular calcium has a “tonic” effect in both the myocardium and vascular smooth muscle.
Catecholamines (eg, norepinephrine, epinephrine, and dobutamine) with beta1 agonist activity regulate intramyocyte calcium levels via the nucleotide cyclic adenosine monophosphate (cyclic AMP) (Fig. 4-2). Beta agonists bind to receptors on the cell surface that are coupled to the intracellular enzyme adenylate cyclase via the stimulatory transmembrane GTP-binding protein. This leads to increased cyclic AMP synthesis, and cyclic AMP, in turn, acts as a second messenger for a series of intracellular reactions resulting in higher levels of intracellular calcium during systole. Less well known is that drugs with only alpha-adrenergic agonist activity also may increase intracellular Ca2+ levels, although by a different mechanism.5,6 Although under investigation, the probable basis for the inotropic effect of alpha-adrenergic drugs is the stimulation of phospholipase C, which catalyzes hydrolysis of phosphatidyl inositol to diacylglycerol and inositol triphosphate (see Fig. 4-2). Both of these compounds increase the sensitivity of the myofilament to calcium, whereas inositol triphosphate stimulates the release of calcium from its intracellular storage site, the sarcoplasmic reticulum. There is still some debate about the mechanism for the inotropic effect of alpha-adrenergic agonists and its significance for the acute pharmacologic manipulation of contractility, but there is little debate about the importance of this mechanism in vascular smooth muscle, where the increase in intracellular calcium stimulated by alpha-adrenergic agonists can increase smooth muscle tone significantly. However, intracellular calcium in vascular smooth muscle is also controlled by cyclic nucleotides.7,8 In contrast to the myocyte, in vascular smooth muscle, cyclic AMP has a primary effect of stimulating the uptake of calcium into intracellular storage sites, decreasing its availability (Fig. 4-3). Thus drugs that stimulate cyclic AMP production (beta agonists) or inhibit its breakdown (phosphodiesterase inhibitors) will cause vasodilation. In addition, cyclic guanosine monophosphate (cyclic GMP) also increases intracellular calcium storage (see Fig. 4-3), decreasing its availability for modulating the interaction of actin and myosin. Several commonly used pharmacologic agents act via cyclic GMP. For example, nitric oxide stimulates the enzyme guanylate cyclase, increasing cyclic GMP levels. Drugs such as nitroglycerin and sodium nitroprusside achieve their effect by producing nitric oxide as a metabolic product. Vasodilation is also produced by “cross-talk” between K+ and Ca2+ fluxes. Decreased levels of ATP, acidosis, and elevated tissue lactate levels increase the permeability of the ATP-sensitive K+ channel. This increased permeability results in hyperpolarization of the cell membrane that inhibits the entry of Ca2+ into the cell. This results in decreased vascular tone.
FIGURE 4-2
Mediators of cardiac contractility. Myocardial contractility is a manifestation of the interaction of actin and myosin, which is facilitated by the binding of calcium to troponin C (TnC). Intercellular calcium levels are controlled by direct flux across the membrane, by cyclic AMP, and by inositol triphosphate (IP3) and diacylglycerol (DAG) produced by the action of phospholipase C (PLC). The synthesis of cyclic AMP is catalyzed by adenylate cyclase (AC), which is activated by binding of agonist to the beta-adrenergic receptor, and its breakdown is catalyzed by phosphodiesterase (PDE), which is inhibited by amrinone and milrinone. The action of PLC is activated by binding of agonist to the alpha-adrenergic receptor.

FIGURE 4-3
Mediators of vascular tone. Cyclic AMP and cyclic GMP increase the uptake of calcium into cellular storage sites in vascular smooth muscle, leading to vasodilation. The synthesis of cyclic GMP is catalyzed by guanylate cyclase, which is activated by nitric oxide (NO), which, in turn, is produced by nitroglycerin (NTG) and sodium nitroprusside (SNP). Excessive vasodilation often is a reflection of other endogenous mediators such as prostaglandins (PGI2, PGE1, PGE2, and PGD2) and thromboxane A2 (Tx A2). Several mediators, such as arachidonic acid (AA), bradykinin, histamine, and substance P, stimulate the release of endothelium-derived relaxing factor (EDRF), which is identified with NO. (Reproduced with permission from Levy JH: Anaphylactic Reactions in Anesthesia and Intensive Care, 2nd ed. Boston, Butterworth-Heinemann, 1992.)

The simplistic overview of pathways of cardiac pharmacology as summarized in Figs. 4-1 through 4-3 also suggests the primary cause of difficulty in the clinical use of the drugs discussed in this chapter. The mechanisms of action for control of heart rate and rhythm, contractility, and vascular tone are interrelated. For example, beta-adrenergic agonists not only increase intracellular calcium to increase contractility, but they also alter K+ currents, leading to tachycardia. Catecholamines not only have beta-adrenergic agonist activity, with inotropic and chronotropic effects, but they also possess alpha-agonist activity, leading to increased intracellular calcium in vascular smooth muscle and vasoconstriction. Phosphodiesterase (PDE) inhibitors like milrinone not only increase contractility by increasing cyclic AMP in the myocyte, but they also cause vasodilation by increasing cyclic AMP in the vasculature. The interplay of the various mechanisms means the clinical art of cardiac surgical pharmacology lies as much in selecting drugs for their side effects as for their primary therapeutic effects.
Arrhythmias are common in the cardiac surgical period. A stable cardiac rhythm requires depolarization and repolarization in a spatially and temporally coordinated manner, and dysrhythmias may occur when this coordination is disturbed. The mechanisms for arrhythmias can be divided into abnormal impulse initiation, abnormal impulse conduction, and combinations of both.9,10 Abnormal impulse initiation occurs as a result of increased automaticity (spontaneous depolarization of tissue that does not normally have pacemaking activity) or as a result of triggered activity from abnormal conduction after depolarizations during phase 3 or 4 of the action potential. Abnormal conduction often involves reentry phenomena, with recurrent depolarization around a circuit owing to unilateral conduction block in ischemic or damaged myocardium and retrograde activation by an alternate pathway through normal tissue. In this simplistic view, it is logical that dysrhythmias could be suppressed by slowing the conduction velocity of ectopic foci, allowing normal pacemaker cells to control heart rate, or by prolonging the action potential duration (and hence refractory period) to block conduction into a limb of a reentry circuit.
A scheme proposed originally by Vaughan Williams and modified subsequently11,12 is used often to classify antidysrhythmic agents, and although alternative schemes describing specific channel-blocking characteristics have been proposed and may be more logical,13 this discussion is organized using the Vaughan Williams system of four major drug categories. In this scheme, class I agents are those with local anesthetic properties that block Na+ channels, class II drugs are beta-blocking agents, class III drugs prolong action potential duration, and class IV drugs are calcium entry blockers. Amiodarone is discussed in detail owing to its expanding role in treating both supraventricular and ventricular arrhythmias and because its use has replaced many of the previously used agents. Because of the efficacy of intravenous amiodarone and its recommendations in Advanced Cardiac Life Support (ACLS) guidelines, many of the older drugs used in cardiac surgery have a historical perspective and are considered briefly.
Although each of the class I agents blocks Na+ channels, they may be subclassified based on electrophysiologic differences. These differences can be explained, to some extent, by consideration of the kinetics of the interaction of the drug and the Na+ channel.14,15 Class I drugs bind most avidly to open (phase 0 of the action potential; see Fig. 4-1) or inactivated (phase 2) Na+ channels. Dissociation from the channel occurs during the resting (phase 4) state. If the time constant for dissociation is long in comparison with the diastolic interval (corresponding to phase 4), the drug will accumulate in the channel to reach a steady state, slowing conduction in normal tissue. This occurs with class Ia (eg, procainamide, quinidine, and disopyramide) and class Ic (eg, encainide, flecainide, and propafenone) drugs. In contrast, for the class Ib drugs (eg, lidocaine and mexiletine), the time constant for dissociation from the Na+ channel is short, the drug does not accumulate in the channel, and conduction velocity is affected minimally. However, in ischemic tissue, the depolarized state is more persistent, leading to greater accumulation of agent in the Na+ channel and slowing of conduction in the damaged myocardium.
Procainamide is a class Ia drug that has various electrophysiologic effects.16 Administration may be limited by the side effects of hypotension and decreased cardiac output.17,18 The loading dose is 20 to 30 mg/min, up to 17 mg/kg, and should be followed by an intravenous infusion of 20 to 80 mg/kg per minute. Because procainamide prolongs action potential duration, widening of the QRS complex often heralds a potential overdose. The elimination of procainamide involves hepatic metabolism, acetylation to a metabolite with antiarrhythmic and toxic side effects, and renal elimination of this metabolite. Thus the infusion rate for patients with significant hepatic or renal disease should be at the lower end of this range.
Class Ib drugs include what is probably the best-known antiarrhythmic agent, lidocaine. As noted, lidocaine is a Na+ channel blocker that has little effect on conduction velocity in normal tissue but slows conduction in ischemic myocardium.14,15 Other electrophysiologic effects include a decrease in action potential duration but a small increase in the ratio of effective refractory period to action potential duration. The exact role of these electrophysiologic effects on arrhythmia suppression is unclear. Lidocaine has no significant effects on atrial tissue, and it is not recommended for therapy in shock-resistant ventricular tachycardia/fibrillation (VT/VF) in the recent Guidelines for Emergency Cardiovascular Care.19 After an initial bolus dose of 1 to 1.5 mg/kg of lidocaine, plasma levels decrease rapidly owing to redistribution to muscle, fat, etc. Effective plasma concentrations are maintained only by following the bolus dose with an infusion of 20 to 50 mg/kg per minute.20 Elimination occurs via hepatic metabolism to active metabolites that are cleared by the kidneys. Consequently, the dose should be reduced by approximately 50% in patients with liver or kidney disease. The primary toxic effects are associated with the central nervous system (CNS), and a lidocaine overdose may cause drowsiness, depressed level of consciousness, or seizures in very high doses. Negative inotropic or hypotensive effects are less pronounced than with most other antiarrhythmics. The other class Ib drugs likely to be encountered in the perioperative period are the oral agents tocainide and mexiletine, which have effects similar to lidocaine.15
The class Ic agents, including flecainide, encainide, and propafenone, markedly decrease conduction velocity.20,21 The Cardiac Arrhythmia Suppression Trial (CAST) study20,21 of moricizine found that although ventricular arrhythmias were suppressed, the incidence of sudden death was greater than with placebo with encainide and flecainide, and these drugs are not in wide use. Propafenone is available for oral use in the United States, but IV form is available in Europe. The usual adult dose is 150 to 300 mg orally every 8 hours. It has beta-blocking (with resulting negative inotropic effects) as well as Na+ channel-blocking activity; lengthens the PR, QRS, and QT duration; and may be used to treat both atrial and ventricular dysrhythmias.15
Beta-receptor-blocking agents are another important group of antiarrhythmic (denoted class II in the Vaughan Williams scheme). However, because of their use as antihypertensive as well as antiarrhythmic agents, they are discussed elsewhere in this chapter, and we will move on to consider bretylium, amiodarone, and sotalol, the class III agents in the Vaughan Williams scheme. These drugs have a number of complex ion channel-blocking effects, but possibly the most important activity is K+ channel blockade.22 Because the flux of K+ out of the myocyte is responsible for repolarization, an important electrophysiologic effect of class III drugs is prolongation of the action potential.23
Ibutilide, dofetilide, sotalol, and bretylium are class III agents. Intravenous ibutilide and oral dofetilide are approved for the treatment of atrial fibrillation (AF) but carry the risk of torsades de pointes.24,25 Sotalol is a nonselective beta-blocker that also has K+ channel-blocking activity.26 In the United States, it is now available for IV and previously for oral administration. The approved indication is for treating life-threatening ventricular arrhythmias, although it is effective against atrial arrhythmias as well. Bretylium is no longer available or recommended in the most recent American Heart Association (AHA) Guidelines for Emergency Cardiovascular Care.19
Calcium entry blockers (class IV in the Vaughan Williams scheme), including verapamil and diltiazem, are antiarrhythmics. In sinoatrial (SA) and atrioventricular (AV) nodal tissues, Ca2+ channels contribute significantly to phase 0 depolarization, and the AV nodal refractory period is prolonged by Ca2+ entry blockade.27,28
This explains the effectiveness of verapamil and diltiazem in treating supraventricular arrhythmias. It is also clear why these drugs are negative inotropes. Both verapamil and diltiazem are effective in slowing the ventricular response to AF, flutter, and paroxysmal supraventricular tachycardia (SVT) and in converting to sinus rhythm.29–31 Verapamil has greater negative inotrope effects than diltiazem; therefore, it is used rarely for supraventricular arrhythmias. The intravenous dose of diltiazem is 0.25 mg/kg, with a second dose of 0.35 mg/kg if the response is inadequate after 15 minutes. The loading dose should be followed by an infusion of 5 to 15 mg/h. Intravenous diltiazem, although useful for rate control, has been replaced by intravenous amiodarone in clinical therapy of SVT and prophylaxis (see Amiodarone).
One of the difficulties of classifying antiarrhythmics by the Vaughan Williams classification is that not all drugs can be incorporated into this scheme. Three examples are digoxin, adenosine, and magnesium, each of which has important uses in the perioperative period.
Digoxin inhibits the Na+, K+-ATPase pump, leading to decreased intracellular K+, a less negative resting membrane potential, increased slope of phase 4 depolarization, and decreased conduction velocity. These direct effects, however, usually are dominated by indirect effects, including inhibition of reflex responses to congestive heart failure (HF) and a vagotonic effect.10,32 The net effect is greatest at the AV node, where conduction is slowed and the refractory period is increased, explaining the effectiveness of digoxin in slowing the ventricular response to AF. The major disadvantages of digoxin are the relatively slow onset of action and many side effects, including proarrhythmia effects, and it is now used rarely for rate control in acute AF because of the advent of IV amiodarone and diltiazem.
Adenosine is an endogenous nucleoside that has an electrophysiologic effect similar to that of acetylcholine. Adenosine decreases AV node conductivity, and its primary antiarrhythmic effect is to break AV nodal reentrant tachycardia.33 An intravenous dose of 100 to 200 μg/kg is the treatment of choice for paroxysmal SVT. Adverse effects, such as bronchospasm, are short-lived because its plasma half-life is so short (1 to 2 seconds). This short half-life makes it ideal for treating reentry dysrhythmia, in which transient interruption can fully suppress the dysrhythmia.
Appropriate acid-base status and electrolyte balance are important because electrolyte imbalance can perturb the membrane potential, leading to arrhythmia generation, as can altered acid-base status, by effects on K+ concentrations and sympathetic tone. Therapy for dysrhythmia should include correction of acid-base and electrolyte imbalances. Magnesium supplementation should be considered.34 Magnesium deficiency is common in the perioperative period, and magnesium administration has been shown to decrease the incidence of postoperative dysrhythmia.35
Intravenous amiodarone has become one of the most administered intravenous antiarrhythmics used in cardiac surgery because of its broad spectrum of efficacy. Amiodarone was developed originally as an antianginal agent because of its vasodilating effects, including coronary vasodilation.36 It has various ion channel-blocking activities.10,29,36 The resulting electrophysiologic effects are complex, and there are differences in acute intravenous and chronic oral administration. Acute intravenous administration can produce decreases in heart rate and blood pressure, but there are minimal changes in QRS duration or QT interval. After chronic use, there may be significant bradycardia and increases in action potential duration in AV nodal and ventricular tissue, with increased QRS duration and QT interval.37–39
Amiodarone is a complex highly lipophilic drug that undergoes variable absorption (35 to 65%) after oral administration and is taken up extensively by multiple tissues with interindividual variation and complex pharmacokinetics.38–40 The short initial context-sensitive half-life after intravenous administration represents drug redistribution. The true elimination half-life for amiodarone is extremely long, up to 40 to 60 days. Because of the huge volume of distribution (~60 L/kg) and a long duration of action, an active metabolite loading period of several months may be required before reaching steady-state tissue concentrations. Further, in life-threatening arrhythmias, intravenous loading often is starting to establish initial plasma levels. Measuring amiodarone plasma concentrations is not useful owing to the complex pharmacokinetics and the metabolites of the parent drug. Plasma concentrations greater than 2.5 mg/L have been associated with an increased risk of toxicity. The optimal dose of amiodarone has not been well characterized and may vary depending on the specific arrhythmias treated. Further, there may be differences in dose requirements for therapy of supraventricular and ventricular arrhythmias.37–40
Because of these distinctive pharmacokinetic properties, steady-state plasma levels are achieved slowly. Oral administration for a typical adult consists of a loading regimen of 80 to 1600 mg/d (in two or three doses) for 10 days, 600 to 800 mg/d for 4 to 6 weeks, and then maintenance doses of 200 to 600 mg/d. For intravenous loading, specific studies will be reviewed, but the recommended dosing is 150 mg given over 10 minutes for acute therapy in an adult, followed first by a secondary loading infusion of 60 mg/h for 6 hours and then by a maintenance infusion of 30 mg/h to achieve a 1000 mg/d dosing.37–40
The electrophysiologic actions of amiodarone are complex and incompletely understood. Amiodarone produces all four effects according to the Vaughan Williams classification. It also has been shown to have use-dependent class I activity, inhibition of the inward sodium currents, and class II activity.10 The antiadrenergic effect of amiodarone, however, is different from that of beta-blocker drugs because it is noncompetitive and additive to the effect of beta-blockers. Amiodarone depresses SA node automaticity, which slows the heart rate and conduction and increases refractoriness of the AV node, properties useful in managing supraventricular arrhythmia. Its class III activity results in increases in atrial and ventricular refractoriness and prolongation of the QTc interval. The effects of oral amiodarone on SA and AV nodal function are maximal within 2 weeks, whereas the effects on ventricular tachycardia (VT) and ventricular refractoriness emerge more gradually during oral therapy, becoming maximal after 10 weeks or more.
The primary indication for amiodarone is VT or fibrillation.40–48 It is also effective for the treatment of atrial dysrhythmias and in treating AF (see Atrial Fibrillation).
Although there are numerous adverse reactions to amiodarone, they occur with long-term oral administration and are rarely associated with acute intravenous administration. The most serious is pulmonary toxicity, which has not been reported with acute administration in a perioperative setting. Some case series have reported an increased risk of marked bradycardia and hypotension immediately after cardiac surgery in patients already on amiodarone at the time of surgery.49,50 Other case-control studies, however, have not reproduced this finding.51 None of the placebo-controlled trials of prophylactic amiodarone for perioperative atrial fibrillation prevention report adverse cardiovascular effects, although bradycardia and hypotension are known side effects.52–56 Case reports and case series of postoperative acute pulmonary toxicity are similarly lacking in the rigor of randomized, controlled methodology.
Intravenous amiodarone is approved for rapid control of recurrent VT or VF. Three randomized controlled trials of patients with recurrent in-hospital, hemodynamically unstable VT or VF with two or more episodes within the past 24 hours who failed to respond to or were intolerant of lidocaine, procainamide, and (in two of the trials) bretylium have been reported.42,44,46 Patients were critically ill with ischemic cardiovascular disease, 25% were on a mechanical ventilator or intraaortic balloon pump before enrollment, and 10% were undergoing cardiopulmonary resuscitation at the time of enrollment. One study compared three doses of IV amiodarone: 525, 1050, and 2100 mg/d.44 Because of the use of investigator-initiated intermittent open-label amiodarone boluses for recurrent VT, the actual mean amiodarone doses received by the three groups were 742, 1175, and 1921 mg/d. There was no statistically significant difference in the number of patients without VT/VF recurrence during the 1-day study period: 32 of 86 (41%), 36 of 92 (45%), and 42 of 92 (53%) for the low-, medium-, and high-dose groups, respectively. The number of supplemental 150-mg bolus infusions of amiodarone given by blinded investigators was statistically significantly less in those randomized to higher doses of amiodarone.
A wider range of amiodarone doses (125, 500, and 1000 mg/d) was evaluated by Sheinman and colleagues, including a low dose that was expected to be subtherapeutic.46 This stronger study design, however, also was confounded by open-label bolus amiodarone injections given by study investigators. There was, however, a trend toward a relationship between intended amiodarone dose and VT/VF recurrence rate (p = .067). After adjustment for baseline imbalances, the median 24-hour recurrence rates of VT/VF, from lowest to highest doses, were 1.68, 0.96, and 0.48 events per 24 hours.
The third study compared two intravenous amiodarone doses (125 and 1000 mg/d) with bretylium (2500 mg/d).42 Once again, the target amiodarone dose ratio of 8:1 was compressed to 1.8:1 because of open-label boluses. There was no significant difference in the primary outcome, which was median VT/VF recurrence rate over 24 hours. For low-dose amiodarone, high-dose amiodarone, and bretylium, these rates were 1.68, 0.48, and 0.96 events per 24 hours, respectively (p = .237). There was no difference between high-dose amiodarone and bretylium; however, more than 50% of patients had crossed over from bretylium to amiodarone by 16 hours.
The failure of these studies to provide clear evidence of amiodarone efficacy may be related to the “active-control study design” used, a lack of adequate statistical power, high rates of supplemental amiodarone boluses, and high crossover rates. Nonetheless, these studies provide some evidence that IV amiodarone (1 g/d) is moderately effective during a 24-hour period against VT and VF.
Although the most effective and rapid treatment of any hemodynamically unstable sustained ventricular tachyarrhythmia is electrical cardioversion or defibrillation, intravenous antiarrhythmic drugs can be used for arrhythmia termination if the VT is hemodynamically stable. The Guidelines for Emergency Cardiovascular Care19 has removed the former recommendation of lidocaine and adenosine use in stable wide QRS tachycardia, now labeled as “acceptable” but not primarily recommended (lidocaine) or not recommended (adenosine). Intravenous procainamide and sotalol are effective, based on randomized but small studies;10 amiodarone is also considered acceptable.19
The Guidelines for Emergency Cardiovascular Care recommends at least three shocks and epinephrine or vasopressin before any antiarrhythmic drug are administered.10,19 No large-scale controlled, randomized studies have demonstrated efficacy for lidocaine, bretylium, or procainamide in shock-resistant VF,10,19 and lidocaine and bretylium are no longer recommended in this setting.19 Two pivotal studies have been reported recently studying the efficacy of agents in acute shock-resistant cardiac arrest.
The Amiodarone in the Out-of-Hospital Resuscitation of Refractory Sustained Ventricular Tachycardia (ARREST) study was randomized, double blind, and placebo controlled. The ARREST study in 504 patients showed that amiodarone 300 mg administered in a single intravenous bolus significantly improves survival to hospital admission in cardiac arrest still in VT or VF after three direct-current shocks (44% vs 34%; p < .03).43 Although the highest survival rate to hospital admission (79%) was achieved when the amiodarone was given within 4 to 16 minutes of dispatch, there was no significant difference in the proportional improvement in the amiodarone group compared with the placebo group when drug administration was delayed (up to 55 minutes). Amiodarone also had the highest efficacy in patients (21% of all study patients) who had a return of spontaneous circulation before drug administration (survival to hospital admission increased to 64% from 41% in the placebo group). Among patients with no return of spontaneous circulation, amiodarone only slightly improved outcome (38% vs 33%).
Dorian performed a randomized trial comparing intravenous lidocaine with intravenous amiodarone as an adjunct to defibrillation in victims of out-of-hospital cardiac arrest.48 Patients were enrolled if they had out-of-hospital ventricular fibrillation resistant to three shocks, intravenous epinephrine, and a further shock or if they had recurrent ventricular fibrillation after initially successful defibrillation. They were randomly assigned in a double-blind manner to receive intravenous amiodarone plus lidocaine placebo or intravenous lidocaine plus amiodarone placebo. The primary end point was the proportion of patients who survived to be admitted to the hospital. In total, 347 patients (mean age 67 ± 14 years) were enrolled. The mean interval between the time at which paramedics were dispatched to the scene of the cardiac arrest and the time of their arrival was 7 ± 3 minutes, and the mean interval from dispatch to drug administration was 25 ± 8 minutes. After treatment with amiodarone, 22.8% of 180 patients survived to hospital admission compared with 12.0% of 167 patients treated with lidocaine (p = .009). Among patients for whom the time from dispatch to the administration of the drug was equal to or less than the median time (24 minutes), 27.7% of those given amiodarone and 15.3% of those given lidocaine survived to hospital admission (p = .05). The authors concluded that compared with lidocaine, amiodarone leads to substantially higher rates of survival to hospital admission in patients with shock-resistant out-of-hospital ventricular fibrillation.
A supraventricular arrhythmia is any tachyarrhythmia that requires atrial or AV junctional tissue for initiation and maintenance. It may arise from reentry caused by unidirectional conduction block in one region of the heart and slow conduction in another, from enhanced automaticity akin to that seen in normal pacemaker cells of the sinus node and in latent pacemaker cells elsewhere in the heart, or from triggered activity, a novel type of abnormally enhanced impulse initiation caused by membrane currents that can be activated and inactivated by premature stimulation or rapid pacing.56–58 Pharmacologic approaches to treating supraventricular arrhythmias, including AF, atrial flutter, atrial tachycardia, AV reentrant tachycardia, and AV nodal reentrant tachycardia, continue to evolve.56–60 Because AF is perhaps the most common arrhythmia after cardiac surgery, this condition is emphasized in detail.
Atrial fibrillation is a common complication of cardiac surgery that increases the length of stay in the hospital with resulting increases in health-care resource utilization.56–60,61 Advanced age, previous AF, and valvular heart operations are the most consistently identified risk factors for this arrhythmia. Because efforts to terminate AF after its initiation are problematic, current interests are directed at therapies to prevent postoperative AF. Most studies suggest that prophylaxis with antiarrhythmic compounds can decrease the incidence of AF, length of hospital stay, and cost significantly. Class III antiarrhythmic drugs (eg, sotalol and ibutilide) also may be effective but potentially pose the risk of drug-induced polymorphic VT (torsades de pointes). Newer intravenous agents including vernakalant were investigated but in the current Food and Drug Administration (FDA) era of drug safety concerns, antiarrhythmic agents are difficult to get approved. Defining which subpopulations benefit most from such therapy is important as older and more critically ill patients undergo surgery. Intravenous sotalol is currently available in the United States.
Amiodarone is also an effective approach for prophylactic therapy of AF. Intravenous amiodarone is an important consideration because loading with oral therapy is often not feasible in part owing to the time required. There also may be added benefits of prophylactic therapies in high-risk patients, especially those prone to ventricular arrhythmias (ie, patients with preexisting HF).
Two studies deserve mention regarding prophylaxis with amiodarone. To determine if IV amiodarone would prevent AF and decrease hospital stay after cardiac surgery, Daoud and colleagues assessed preoperative prophylaxis in 124 patients who were given either oral amiodarone (64 patients) or placebo (60 patients) for a minimum of 7 days before elective cardiac surgery.62 Therapy consisted of 600 mg amiodarone per day for 7 days and then 200 mg/d until the day of discharge from the hospital. The preoperative total dose of amiodarone was 4.8 ± 0.96 g over 13 ± 7 days. Postoperative AF occurred in 16 of the 64 patients in the amiodarone group (25%) and 32 of the 60 patients in the placebo group (53%). Patients in the amiodarone group were hospitalized for significantly fewer days than were patients in the placebo group (6.5 ± 2.6 vs 7.9 ± 4.3 days; p = .04). Total hospitalization costs were significantly less for the amiodarone group than those for the placebo group ($18,375 ± $13,863 vs $26,491 ± $23,837; p = .03). Guarnieri and colleagues evaluated 300 patients randomized in a double-blind fashion to IV amiodarone (1 g/d for 2 days) versus placebo immediately after open-heart surgery.54 The primary end points of the trial were incidence of AF and length of hospital stay. AF occurred in 67 of 142 (47%) patients on placebo versus 56 of 158 (35%) on amiodarone (p = .01). Length of hospital stay for the placebo group was 8.2 ± 6.2 days, and 7.6 ± 5.9 days for the amiodarone group. Low-dose IV amiodarone was safe and effective in reducing the incidence of AF after heart surgery but did not significantly alter length of hospital stay.
In summary, AF is a frequent complication of cardiac surgery. Many cases can be prevented with appropriate prophylactic therapy. Beta-adrenergic blockers should be administered to most patients without contraindication. Prophylactic amiodarone should be considered in patients at high risk for postoperative AF. The lack of data on cost-benefits and cost-efficiency in some studies may reflect the lack of higher-risk patients in the study. Patients who are poor candidates for beta-blockade may not tolerate sotalol, whereas amiodarone does not have this limitation. Additional studies also need to be performed to better assess the role of prophylactic therapy in off-pump cardiac surgery.
Some depression of myocardial function is common after cardiac surgery.63–65 The etiology is multifactorial—preexisting disease, incomplete repair or revascularization, myocardial edema, postischemic dysfunction, reperfusion injury, etc—and usually is reversible. Adequate cardiac output usually can be maintained by exploiting the Starling curve with higher preload, but often the cardiac function curve is flattened, and it is necessary to use inotropic agents to maintain adequate organ perfusion.
The molecular basis for the contractile property of the heart is the interaction of the proteins actin and myosin, in which chemical energy (in the form of ATP) is converted into mechanical energy. In the relaxed state (diastole), the interaction of actin and myosin is inhibited by tropomyosin, a protein associated with the actin-myosin complex. With the onset of systole, Ca2+ enters the myocyte (during phase 1 of the action potential). This influx of Ca2+ triggers the release of much larger amounts of Ca2+ from the sarcoplasmic reticulum. The binding of Ca2+ to the C subunit of the protein troponin interrupts inhibition of the actin-myosin interaction by tropomyosin, facilitating the hydrolysis of ATP with the generation of a mechanical force. With repolarization of the myocyte and completion of systole, Ca2+ is taken back up into the sarcoplasmic reticulum, allowing tropomyosin to again inhibit the interaction of actin and myosin with consequent relaxation of contractile force. Thus inotropic action is mediated by intracellular Ca2+.66 A novel drug, levosimendan, currently under clinical development in the United States but approved in several countries, increases the sensitivity of the contractile apparatus to Ca2+,67 whereas the positive inotropic agents available for clinical use achieve their end by increasing intracellular Ca2+ levels.
The first drug to be considered is simply Ca2+ itself. In general, administration of calcium will increase the inotropic state of the myocardium when measured by load-independent methods, but it also will increase vascular tone (afterload) and impair diastolic function. In addition, the effects of calcium on myocardial performance depend on the plasma Ca2+ concentration. Ca2+ plays important roles in cellular function, and the intracellular Ca2+ concentration is highly regulated by membrane ion channels and intracellular organelles.68,69 If the extracellular Ca2+ concentration is normal, administration of Ca2+ will have little effect on the intracellular level and less pronounced hemodynamic effects. On the other hand, if the ionized plasma calcium concentration is low, exogenous calcium administration may increase cardiac output and blood pressure.70 It also should be realized that even with normal plasma Ca2+ concentrations, administration of Ca2+ may increase vascular tone, leading to increased blood pressure but no change in cardiac output. This increased afterload, as well as the deleterious effects on diastolic function, may be the basis of the observation that Ca2+ administration can blunt the response to epinephrine.71 Routine use of Ca2+ at the end of bypass should be tempered by the realization that Ca2+ may have little effect on cardiac output while increasing systemic vascular resistance, although this in itself may be of importance. If there is evidence of myocardial ischemia, Ca2+ administration may be deleterious because it may exacerbate both coronary spasm and the pathways, leading to cellular injury.72,73
Digoxin, although not effective as acute therapy for low-cardiac-output syndrome in the perioperative period, nevertheless well illustrates the role of intracellular Ca2+. Digoxin functions by inhibiting Na+, K+-ATPase, which is responsible for the exchange of intracellular Na+ with extracellular K+.3,4 It is thus responsible for maintaining the intracellular/extracellular K+ and Na+ gradients. When it is inhibited, intracellular Na+ levels increase. The increased intracellular Na+ is an increased chemical potential for driving the Ca2+/Na+ exchanger, an ion exchange mechanism in which intracellular Na+ is removed from the cell in exchange for Ca2+. The net effect is an increase in intracellular Ca2+ with an enhancement of the inotropic state.
The most commonly used positive inotropic agents are the beta-adrenergic agonists. The beta1 receptor is part of a complex consisting of the receptor on the outer surface of the cell membrane and membrane-spanning G proteins (so named because they bind GTP), which in turn stimulate adenylate cyclase on the inner surface of the membrane, catalyzing the formation cyclic adenosine monophosphate (cyclic AMP). The inotropic state is modulated by cyclic AMP via its catalysis of phosphorylation reactions by protein kinase A. These phosphorylation reactions “open” Ca2+ channels on the cell membrane and lead to greater release and uptake of Ca2+ from the sarcoplasmic reticulum.3,4
There are many drugs that stimulate beta1 receptors and have a positive inotropic effect, including epinephrine, norepinephrine, dopamine, isoproterenol, and dobutamine, the most commonly used catecholamines in the perioperative period. Although there are differences in their binding at the beta1 receptor, the most important differences between the various catecholamines are their relative effects on alpha- and beta2 adrenergic receptors. In general, alpha receptor stimulation on the peripheral vasculature causes vasoconstriction, whereas beta2 receptor stimulation leads to vasodilation (see the discussion elsewhere in this chapter). For some time it was believed that beta2 and alpha receptors were found only in the peripheral vasculature, as well as in a few other organs, but not in the myocardium. However, alpha receptors are also found in the myocardium and mediate a positive inotropic effect.5,6 The mechanism for this positive inotropic effect is probably the stimulation of phospholipase C, leading to hydrolysis of phosphatidyl inositol to diacylglycerol and inositol triphosphate, compounds that increase Ca2+ release from the sarcoplasmic reticulum and increase myofilament sensitivity to Ca2+. It is also possible that alpha-adrenergic agents increase intracellular Ca2+ by prolonging action potential duration by inhibition of outward K+ currents during repolarization or by activating the Na+/H+ exchange mechanism, increasing intracellular pH and increasing myofilament sensitivity to Ca2+. Just as the exact mechanism is uncertain, the exact role of alpha-adrenergic stimulation in control of the inotropic state is unclear, although it is apparent that onset of the effect is slower than that of beta1 stimulation.
Besides the discovery of alpha receptors in the myocardium, beta2 receptors are present in the myocardium.74 The fraction of beta2 receptors (compared with beta1 receptors) is increased in chronic HF, possibly explaining the efficacy of drugs with beta2 activity in this setting. This phenomenon is part of the general observation of beta1 receptor downregulation (decrease in receptor density) and desensitization (uncoupling of effect from receptor binding) that is observed in chronic HF.75 Interestingly, it has been demonstrated in a dog model that this same phenomenon occurs with cardiopulmonary bypass (CPB).76 In this situation, a newer class of drugs, the phosphodiesterase inhibitors, may be of benefit. These drugs, typified by the agents available in the United States, amrinone and milrinone, increase cyclic AMP levels independently of the beta receptor by selectively inhibiting the myocardial enzyme responsible for the breakdown of cyclic AMP.3,4
In clinical use, selection of a particular inotropic agent usually is based more on its side effects than its direct inotropic properties. Of the commonly used catecholamines, norepinephrine has alpha and beta1 but little beta2 activity and is both an inotrope and a vasopressor. Epinephrine and dopamine are mixed agonists with alpha, beta1, and beta2 activities. At lower doses, they are primarily inotropes and not vasopressors, although vasopressor effects become more pronounced at higher doses. This is especially true for dopamine, which achieves effects at higher doses by stimulating the release of norepinephrine.77 Dobutamine is a more selective beta1 agonist, in contrast to isoproterenol, which is a mixed beta agonist. Selection of a drug depends on the particular hemodynamic problem at hand. For example, a patient with depressed myocardial function in the presence of profound vasodilation may require a drug with both positive inotropic and vasopressor effects, whereas a patient who is vasoconstricted may benefit from some other choice. Recent studies report that in patients with myocardial infarction (MI) who develop cardiogenic shock, dopamine increases mortality compared to norepinephrine.78,79 On the basis of multiple considerations, we recommend an empiric approach to selecting inotropic agents with careful monitoring of the response to the drug and selection of the agent that achieves the desired effect.
Clinical experience suggests that phosphodiesterase inhibitors are effective when catecholamines do not produce an acceptable cardiac output. Milrinone is the phosphodiesterease most used, and amrinone is no longer available. Enoximone, a similar agent with different pharmacokinetic properties, was used in Europe but not available in the United States. All agents increase contractility with minimal effect on heart rate, and both are vasodilators. There is significant venodilation, as well as pulmonary and systemic vasodilation, and maintaining adequate preload is important in avoiding hypotension.80–82 Administering the loading dose over 15 to 30 minutes may also attenuate possible hypotension, or alternately starting an infusion alone without loading is an alternative method. Plasma levels drop rapidly after a loading dose because of redistribution, and the loading dose should be followed immediately by a continuous infusion.82–84 Because of their longer half-lives, it is rather more difficult to readily titrate plasma levels than with catecholamines (which have plasma half-lives of a few minutes).
Phosphodiesterase inhibitors, specifically milrinone, facilitate separation from CPB with biventricular dysfunction and are used for treating low-cardiac-output syndrome after cardiac surgery.82–86 Doolan and colleagues also demonstrated that milrinone, in comparison with placebo, significantly facilitated separation of high-risk patients from CPB.87 Despite the extensive use of pharmacologic therapy, there are no convincing data to support a distinct inotropic or vasodilator drug-based therapy as a superior solution to treat biventricular dysfunction and/or potentially reduce mortality in hemodynamically unstable patients with cardiogenic shock or low cardiac output, especially complicating an acute MI.88
Levosimendan is a class of drugs known as calcium sensitizers. The molecule is a pyridazinone-dinitrile derivative with additional action on ATP-sensitive potassium channels.67,89,90 Levosimendan is used intravenously for treating decompensated HF because it increases contractility and produces antistunning effects without increasing myocardial intracellular calcium concentrations or prolonging myocardial relaxation. Levosimendan also causes coronary and systemic vasodilation. In patients with HF, IV levosimendan reduced worsening HF or death significantly. IV levosimendan significantly also increased cardiac output and decreased filling pressure in decompensated HF in large, double-blind, randomized trials and after cardiac surgery in smaller trials. Levosimendan is well tolerated without arrhythmogenicity. In addition to sensitizing troponin to intracellular calcium, levosimendan inhibits phosphodiesterase III and open ATP-sensitive potassium channels (KATP), which may produce vasodilation. Unlike currently available intravenous inotropes, levosimendan does not increase myocardial oxygen use and has been used effectively in beta-blocked patients. Levosimendan does not impair ventricular relaxation. Clinical studies have demonstrated short-term hemodynamic benefits of levosimendan over both placebo and dobutamine. Although large-scale, long-term morbidity and mortality data are scarce, the Levosimendan Infusion versus Dobutamine in Severe Low-Output Heart Failure (LIDO) study suggested a mortality benefit of levosimendan over dobutamine. Clinical studies comparing levosimendan with other positive inotropes, namely, milrinone, are lacking. Currently, this agent is being evaluated in a clinical trial in North America for patients with left ventricular dysfunction undergoing CPB (https://clinicaltrials.gov/ct2/show/NCT02025621?term=levosimendan&rank=14).
Despite their common use after cardiac surgery, there have been relatively few comparative studies of inotropic agents in the perioperative period. In 1978, Steen reported the hemodynamic effects of epinephrine, dobutamine, and epinephrine immediately after separation from CPB.91 The largest mean increase in cardiac index was achieved with dopamine at 15 mcg/kg per minute. However, it should be noted that the only epinephrine dose studied was 0.04 mcg/kg per minute. In a later comparison of dopamine and dobutamine, Salomon concluded that dobutamine produced more consistent increases in cardiac index, although the hemodynamic differences were small, and all patients had good cardiac indices at the onset of the study.92 Fowler also found insignificant differences in the hemodynamic effects of dobutamine and dopamine, although they reported that coronary flow increased more in proportion to myocardial oxygen consumption with dobutamine.93 Although neither of these groups reported significant increases in heart rate for either dopamine or dobutamine, clinical experience has been otherwise. This is supported by a study by Sethna, who found that the increase in cardiac index with dobutamine occurs simply because of increased heart rate, although they found that myocardial oxygen was maintained.94 Butterworth subsequently demonstrated that the older and much cheaper agent, epinephrine, effectively increased stroke volume without as great an increase in heart rate as dobutamine.95 More recently, Feneck compared dobutamine and milrinone and found them to be equally effective in treating low-cardiac-output syndrome after cardiac surgery.96 This study was a comparison of two drugs, and the investigators emphasized that the most efficacious therapy is probably a combination of drugs. In particular, phosphodiesterase inhibitors require the synthesis of cyclic AMP to be effective, and thus use of a combination of a beta1-adrenergic agonist and a phosphodiesterase inhibitor would be predicted to be more effective than either agent alone.
Finally, while global hemodynamic goals (ie, heart rate, blood pressure, filling pressures, and cardiac output) may be achieved with inotropic agents, this does not guarantee adequate regional perfusion, in particular renal and mesenteric perfusion. So far there have been few investigations of regional perfusion after cardiac surgery. There has been more interest in regional (especially mesenteric) perfusion in the critical care medicine literature, and some of the studies may be relevant to postoperative care of the cardiac surgical patient. Two studies have indicated that epinephrine may impair splanchnic perfusion, especially in comparison with combining norepinephrine and dobutamine.97,98 Norepinephrine alone has variable effects on splanchnic blood flow in septic shock,99 although adding dobutamine can improve splanchnic perfusion significantly when blood pressure is supported with norepinephrine.98 Low-dose dopamine improves splanchnic blood flow,100 but there is evidence that dopamine in higher doses impairs gastric perfusion.101 The relevance of these studies of septic patients for the cardiac surgical patient is unclear, although there are similarities between the inflammatory responses to CPB and to sepsis.
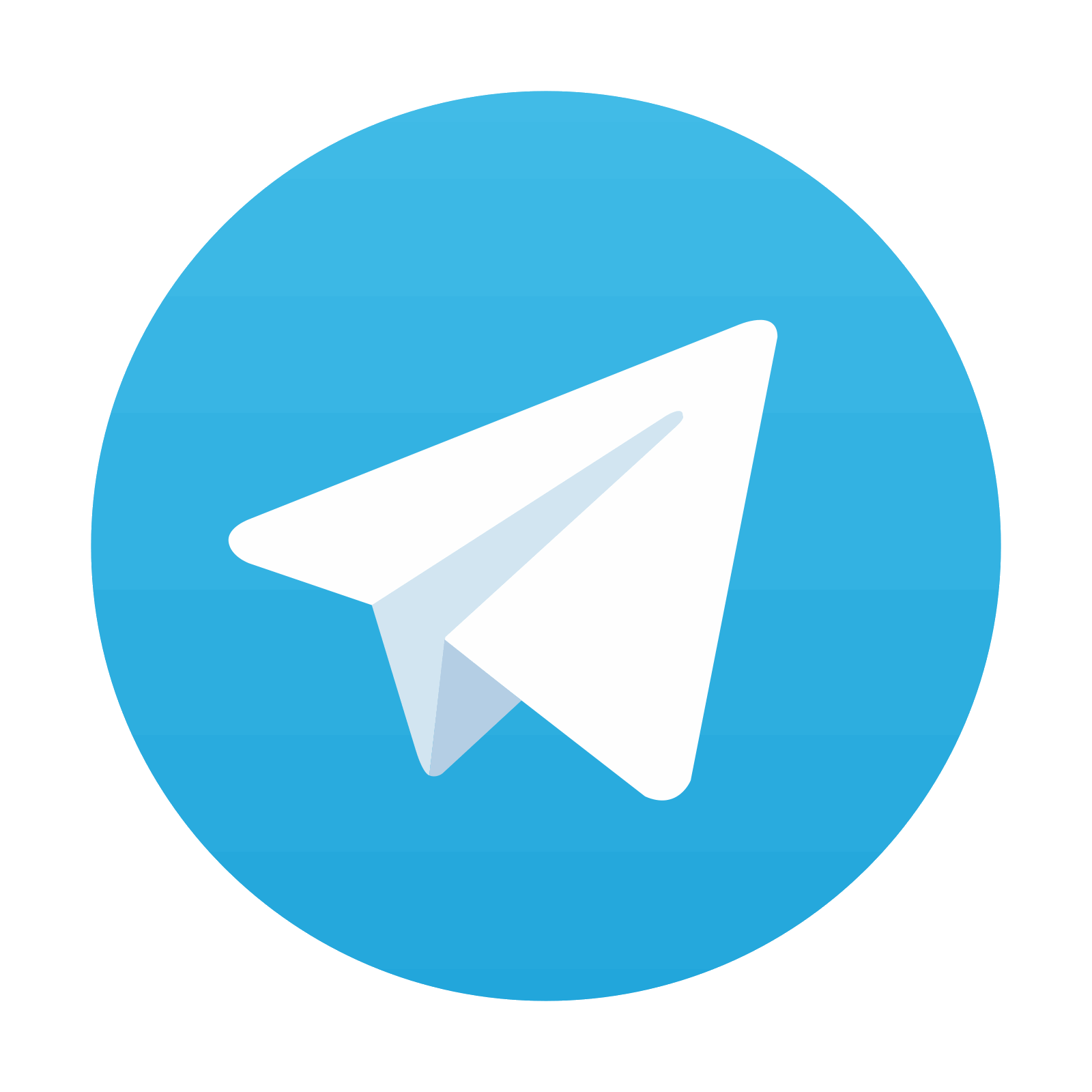
Stay updated, free articles. Join our Telegram channel
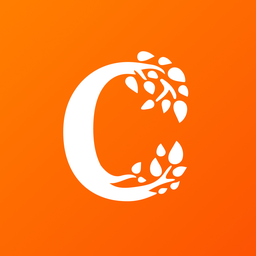
Full access? Get Clinical Tree
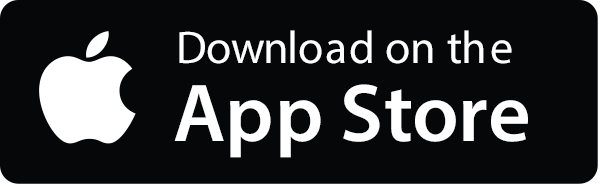
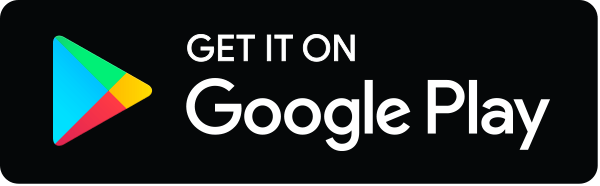