The changing needs of cardiologists and patients have led to an unprecedented evolution in nuclear cardiology instrumentation. Modern instrumentation has a wide range of collimation, attenuation correction (AC) options, and many of the newest systems no longer relying on the Anger gamma camera design. Solid-state detector technologies and new aperture designs are commercially available and in widespread use. Hybrid SPECT and PET systems now have transmission imaging options for AC using x-ray CT, radionuclide, or novel fluorescence x-ray sources.
The changes in instrumentation have been driven by revolutionary changes in the delivery of health care. In particular, there has been a shift from office-based nuclear cardiology services to hospital-based imaging. According to MedAxiom, respondents reported a dramatic shift in providers from private practices to integrated practices. In 2008, only 11% of MedAxiom respondents reported being in an integrated group (e.g., integrated into a hospital environment). By 2012, almost 60% of respondents reported practicing within an integrated practice.1 The integration of cardiovascular imaging into the hospital setting has significantly altered the kinds of instrumentation available to the cardiologist. This access has provided clinicians the capability for routine AC, neuronal imaging, absolute blood flood, sarcoid imaging, amyloid imaging, metabolic imaging (glucose and fatty acid tracers) and other advanced molecular imaging techniques. This chapter will describe the instrumentation that is commonly used in nuclear cardiology and explain some of the important advances in instrumentation that can improve image quality and reduce radiation exposure.
Hal Anger, with his team at the University of California, Berkeley had worked with the rectilinear scanners and pin-hole camera designs. In 1957, this group changed the design to allow for a uniform magnification and resolution across the field of view with increased system sensitivity. The first “Anger” scintillator camera consisted of a 4-in sodium iodine crystal optically coupled to 7 photomultiplier tubes (PMTs).2,3
Gamma camera design has evolved significantly since 1957 with improvements in resolution, sensitivity, and energy discrimination, however the conceptual design of the Anger camera is remarkably unchanged. The “Anger” gamma camera consists of (see Fig. 4-1):
Focusing, multi-hole collimator: The focusing collimator is placed in front of the other camera components. The image is formed by excluding photons not traveling along a particular path, for example, perpendicular to the camera face or holes focusing on a point or line.
Scintillation crystal: A material that will luminesce with optical photons when excited by ionizing radiation.
PMT array: Electronics for capturing the photons produced by the scintillator crystal and converting those photons into an electronic pulse.
Analog-to-digital conversion hardware: A digitization board converts the analog pulse-height information into a position and energy for each photon event.
Unlike optical photons, gamma photons cannot be focused with either reflection or refraction. “Focusing” for gamma rays is typically performed by preferentially accepting only those photons traveling in a particular direction. The use of collimation dramatically reduces photon sensitivity by absorbing almost 99.99% of all photons entering the camera. The most common collimator used in SPECT is a parallel-hole collimator. These collimators function by using an array of parallel holes in a block of attenuating material such as lead or tungsten (see Fig. 4-2). There are some less common geometry collimators that are specific to various cardiac application such as fan beam collimation that focus on a line at a fixed distance from the collimator and variable distance collimator.4
The use of collimation instead of the lenses that are used in conventional photography, leads to some interesting differences in nuclear imaging:
The use of collimation results in a loss of resolution with increasing source distance from the detector surface (see Fig. 4-3).5
Sensitivity is constant with distance. Specifically, the number of counts/second recorded is independent of distance, so long as the loss of resolutions does not result in counts missing the detector entirely.
The field of view for parallel-hole collimation does not change with distance.
The resolution of a gamma camera depends on the diameter and length of the holes in the collimating material. “High-resolution” collimators tend to have a longer hole length, smaller hole diameter, and thinner septa compared with “general-purpose” or “all-purpose” collimators.6
Parallel-hole collimation is generally favored but most nuclear laboratories because of the consistency of the resolution with patient positioning, camera rotation, and protocol variation.7
Fan-beam geometry allows radiation emitted from a source at a specific distance to pass through the collimator without interaction and reach the crystal surface.8 A hybrid design combining both fan-beam properties in the center of the field of view and a gradual approach to parallel-hole design toward the edge of the field of view has been implemented on a dual detector Anger-based SPECT system for cardiac SPECT.4,9 This design improves the geometric component of system sensitivity by a factor of 2 over parallel geometry and minimizes truncation of projections near the edge of the field of view.
When a gamma photon is absorbed by the scintillation crystal, a burst of secondary optical photons is then released. An array of PMTs, positioned behind the crystal, detects and converts the light pulse into an electric pulse that is digitized.
The digitization process is a mathematical weighting of the pulses from all PMTs detecting the scintillation produces X, Y (spatial location), and Z (energy) values. The energy value is tested by a discrimination circuit that will exclude the signal if it is not within the permitted energy range (referred to as the “energy window”).
Energy resolution has an important role in differentiating between scatter and photopeak photons. When gamma rays scatter off of electrons in the media, they release some of their energy to the electrons (referred to as the recoil electron). As a result of scattering of the electrons, the energy of the scattered photon is reduced. This process of photons elastically scattering off of electrons in the media is referred to as Compton scattering. Compton scattering degrades the images quality by reducing the contrast resolution and spatial resolution of the image.
System sensitivity is another key imaging performance measure expressed in units of counts/min/μCi (cpm/μCi) of Tc-99m. It is dependent on the length and diameter of the holes in the collimator. Higher system sensitivity collimators allow more photons to pass through the collimator at the expense of spatial resolution. Conversely, higher-resolution collimators allow fewer photons to pass through the collimator by restricting the acceptance angle of the collimator holes. This reduction in acceptance angle improves the spatial resolution of the system.
To produce tomographic images of a patient, a series of projections around the patient is needed. The most common approach is to mount one or more Anger cameras on a rotating gantry. Early designs for the gantry involved a single camera head that could be rotated around the patient on a fork mount. Because these systems were also used for planar imaging, the camera head could be rotated around two axes.10 With the increase use of SPECT and the challenge of maintaining an accurate center of rotation led to near universal use of the single axis of rotation gantry for cardiac studies.
The most common design in use today for cardiac SPECT is a dual-headed gamma camera with 90 degrees of separation between each camera head (see Fig. 4-4). To complete a tomographic study, the camera only needs to complete a 90-degree arc, and camera heads rotate simultaneously, thus saving image acquisition time. This geometry offers an excellent combination of a wide field of view, scanning efficiency, and simplicity. Most systems also offer the option to acquire a noncircular orbit to minimize the distance between the camera and the patient. This has the effect of improving the resolution by up to 1.5 to 2.5 mm.11
Several solid-state detector-based systems are now available commercially.12–14 These systems have a crystal layer placed over an array of semiconductor units that collect the scintillation light directly forming a net electronic charge in each unit that is proportional to the incident scintillation light. Because of the more direct coupling of crystal and detectors, the energy and location are more easily determined. Common solid-state crystals are cadmium-zinc telluride (CZT) and cesium-iodide (CsI) due to their physical and optical emission properties.15 A lead or tungsten collimator is also used over the crystal surface for localization of incident photons as with the Anger approach. In comparison to Anger systems, solid-state detectors have superior energy resolution compared with the NaI(Tl) crystals. Thus, solid-state detectors intrinsically have greater contrast resolution. System sensitivity of the solid-state detectors is modestly (10–15%) lower than Anger systems.16 However, this decrease in acquisition efficiency is offset by the use of multiarrays of detectors encompassing greater angular range for detection about the patient that improves the efficiency for detection.
More recently, several alternatives to the Anger gamma camera have been introduced. One such system utilizes nine independent scanning detectors to concentrate the imaging on a small field of view, centered on the heart12 (D-SPECT, Spectrum Dynamics, Haifa, Israel). The advantage of this system is the ability of the scanner to concentrate the acquisition on the myocardium. This has the effect of preferentially improving the system’s SPECT sensitivity in the region of the heart (see Fig. 4-5). The D-SPECT system does not have the same quality control procedures as a conventional Anger SPECT system. Daily quality control (QC) consists of three QC steps: (1) global homogeneity, (2) regional homogeneity, and (3) field of view.17 These must be performed to insure optimal image quality.
Several studies have been performed to investigate the potential for this scanner for ultra–low-dose, stress first imaging.18 In a study of 284 patients (208 with coronary angiography, 76 low likelihood) populations were split into a 128 standard stress dose (10.2 ± 0.5 mCi) patients and 156 low stress dose (5.9 ± 1.2 mCi). In that study there was no significant difference between sensitivity, specificity, and accuracy between the low dose and standard dose studies (86.1%, 76.6%, and 81.4%; and 90.6%, 78.1%, and 84.4%, respectively, p = ns). This implied that for those patients that only required a stress study, the radiation dose was as 1.7 ± 0.3 mSv.
In the multipinhole aperture approach,13,19 individual “pinholes” are placed strategically on the surface of a solid lead structure on each detector such that photons may only pass through the apertures to reach the underlying detectors (see Fig. 4-6). This approach permits the images to be acquired without gantry rotation as with conventional systems. The system sensitivity (per unit activity) compared with conventional Anger-based systems is approximately five times that of conventional SPECT imaging.
One such system consisting of 19 pinholes has become available to cardiologists (Discovery NM 530c, General Electric). This system projects onto 4 solid-state CZT pixilated detector. This system has the advantage that there are no moving parts and is 5 to 10 times more sensitivity than conventional Anger systems.20
Sensitivity and specificity of the Discovery NM 530c were studied in a population of 160 patients imaged myocardial perfusion patients using a weight-adjusted rest/stress Tc-99m-sestamibi study. In that study, the automated, computer results showed higher specificity (59–67% vs. 27–60%) and lower sensitivity (71–72% vs. 79–93%) than the visual reads.21 This would suggest that the appearance of these images may be different than conventional images, however the differences are predictable. It is likely that with experience, visual interpretation should approach computer-generated results.
AC is employed in cardiac SPECT to compensate for image artifacts that can result from absorption of photons by the soft tissue of the patient.22–24 AC requires an anatomical map as additional information for the reconstruction algorithm to compensate for this loss of signal. Two transmission imaging approaches commonly used to measure the patient anatomy are as follows:
Radionuclide sources: These sources can acquire transmission data simultaneously or sequentially with the emission scan while the x-ray–based approaches acquire images only in sequential mode.8,22 These approaches typically have a very low radiation dose (0.03 mSv).25
X-ray–based CT systems: X-ray CT images also have spatial resolution that is on the order of 10 times greater than the emission images. X-ray CT images can be temporally gated with ECG signals for AC while radionuclide source methods are generally not acquired with ECG gating.23
Scanning line sources using Gd-153 (100 keV, T1/2 = 273 days) are the most commonly used radionuclide source method for SPECT AC.8,22 These systems use an external source of radiation to project gamma rays through the patient to the detector on the far side of the patient. To create a transmission reconstruction of the attenuating medium, two scans are needed: a blank scan of the line source without the patient present, and a scan with the patient present. The ratio of the scan divided by the blank scan is then used to create a patient-specific map of the attenuation.
One advantage line source AC has over CT-based AC is that it can be performed simultaneous to the emission study. This improves laboratory efficiency and removes the possibility of misregistration between the transmission and emission datasets. Another advantage of using a line source AC is the elimination of common CT artifacts such as breathing artifacts, metal artifacts, etc.
The greatest challenge of using line source AC is acquiring sufficient counts to perform a transmission reconstruction. It is important for quality of AC imaging to maintain the strength of the radionuclide sources by periodic replenishment as recommended by the manufacturer.26 As the sources decay, the acquisition time must be extended proportionally to maintain image quality, which can decrease patient convenience and laboratory efficiency. Compromised quality of the attenuation map may result from image noise otherwise, introducing artifacts in the attenuation maps resulting in compromised accuracy or artifacts in the corrected images.27
The high spatial resolution of x-ray CT images has significant implications for AC quality. CT-based AC is similar to line source AC in that a patient-specific map of the patient’s anatomy is used to compensate for the influence of soft tissue attenuation. Though this process on its surface is similar to line source AC, there are several important differences:
X-ray CT-based transmission imaging is by necessity performed sequentially to the emission study. This has a negative impact on laboratory efficiency. This also can introduce artifacts that are a result of misregistration between the transmission and emission datasets.
X-ray CT-based images are recorded in Hounsfield units. An algorithm is needed to translate these units into attenuation coefficients.
X-ray CT-based AC typically has higher radiation dose for the patient.28 Every effort must be made to minimize the radiation from the CT portion of the study.
Proper AC requires the attenuation map images be aligned in all dimensions with the SPECT images.29 As the CT images may be acquired over a single phase, and the emission images over multiple phases, this is not always easily performed. As a result, the attenuation map boundaries of the CT images are much sharper compared with the radionuclide images in the same patient. Misalignment by as little as 1 cm may produce artifacts with severity similar to true perfusion defects. The linear attenuation coefficients (or Hounsfield units), which comprise the attenuation map pixel values, vary greatly in the thorax compared with other regions of the body where lung tissue values for 140 keV (Tc-99m) are almost 10 times less than those for the myocardium. Whenever CT-based AC is applied, images must be carefully inspected for misregistration and corrected as necessary.30
One application that has been suggested is to use diagnostic quality CT in conjunction with myocardial perfusion to assess the presence of coronary calcium in patients with no known history of CAD. In a study of 1,126 asymptomatic patients with a quantitative coronary calcium score, it was demonstrated that the use of coronary calcium assessment in conjunction with myocardial perfusion assessment independently improved the prediction of future cardiac events.31 There have also been smaller studies that suggest that the combination of SPECT with CT angiographic result could provide some added clinical value, additional research is needed to establish optimal clinical scenarios and justification for the additional procedure and radiation dose.32
In conventional parallel-hole SPECT imaging, multiple projection images of the patient are taken at uniformly spaced angles to obtain a three-dimensional (3D) image. Iterative algorithms, described below, may include additional information about the physics of the imaging process, including spatial resolution, noise, and attenuation properties to improve the accuracy of reconstruction.33–35
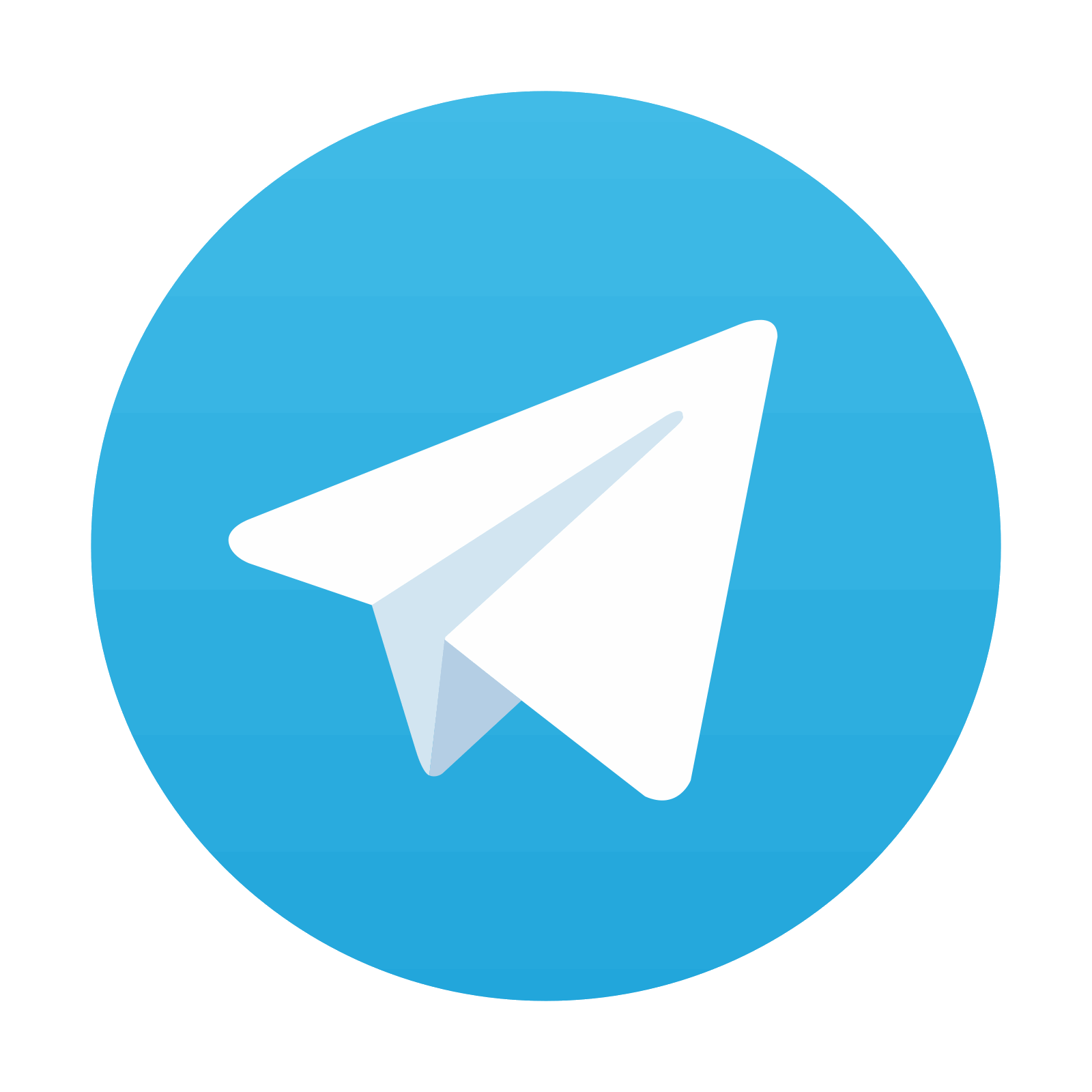
Stay updated, free articles. Join our Telegram channel
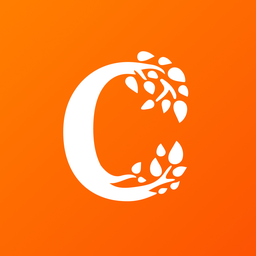
Full access? Get Clinical Tree
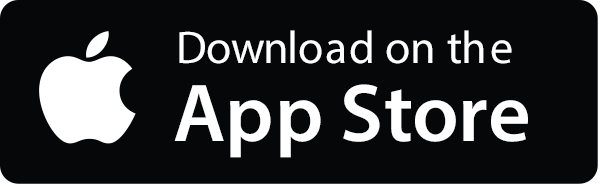
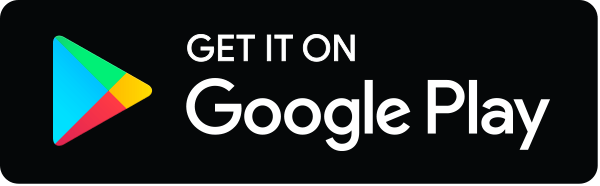