Fig. 6.1
The miRNA biogenesis pathway. In the nucleus, pri-miRNAs are transcribed by RNA polymerase II as capped (m7G, 7-methylguanosine-cap) and polyadenylated transcripts. The pri-miRNA can consist of one pre-miRNA or a cluster of pre-miRNAs. Processing of the pri-miRNA is catalyzed by the micro-processor complex (Drosha and DGCR8) inside the nucleus. Following micro-processing, the pre-miRNA is transported out of the nucleus by RAN-GTP dependent transporter Exportin 5. In the cytoplasm, the pre-miRNA is processed by Dicer, assisted by TRBP and PACT. The generated miRNA duplex is loaded into the miRISC, composed of an Ago protein (AGO1-4) and GW182. This is followed by guide strand selection. The mature miRNA inside the miRISC (red) guides the complex to the target mRNA
6.1.3 microRNA Target Recognition
The miRNA within the miRISC targets mRNA molecules to induce miRNA-mediated translational repression as well as miRNA-mediated mRNA decay. Target interaction between mRNAs and miRNAs involves seed-pairing between nucleotides of both strands. Recognition of the mRNA target does not require perfect complementarity. In mammals, mRNA target recognition generally involves mRNA sites in the 3′-UTR that base-pair with approximately 7 nucleotides near the 5′-end of the miRNA. These miRNA nucleotides are termed ‘the seed sequence’ (Lewis et al. 2005). However, miRNA target sites in the coding sequence of mRNAs have also been discovered and miRNA target sites that cannot be explained by this canonical seed-pairing model also exist. This makes miRNA target identification a very difficult task (Thomas et al. 2010). Even though the miRNA guides the miRISC, the protein components of the miRISC execute the silencing of target mRNAs.
6.1.4 microRNA-Induced Silencing Complex
The first miRNA, lin-4, was reported to inhibit translation of lin-14 mRNA without destabilizing the mRNA molecule (Lee et al. 1993). There have been several studies using C. elegans and mammalian cell cultures that only observed translational repression at the initiation of translation (Ding and Grosshans 2009; Bhattacharyya et al. 2006). In contrast, others reported miRNA-mediated deadenylation, decapping, and subsequent decay of miRNA-target molecules (Eulalio et al. 2009). Over the past few years, there has been growing evidence for translational repression as well as mRNA decay by miRNAs (Huntzinger and Izaurralde 2011).
Recent studies on translational repression of miRNAs propose that the miRISC interferes with the function of the cap-binding complex. Mammalian mRNAs exist as capped and polyadenylated transcripts that are circularized by protein complexes interacting with the 5′-cap structure (comprising the cap-binding complex) and the 3′-poly(A)-tail. Circularization of mRNAs is necessary for efficient translation and interfering with the cap-binding complex can result in loss of circularization and subsequent translational repression (Mathonnet et al. 2007; Fukao et al. 2014). In addition, GW182 proteins are known to interact with poly(A) binding proteins (PABP ). Recently, it was found that interaction between GW182 and PABP leads to disassociation of PABP from the mRNA molecule. This can cause disruption of mRNA circularization and inhibit efficient translation, hereby facilitating translational repression (Zekri et al. 2013).
A second mechanism by which miRNAs regulate target mRNAs is mRNA destabilization and subsequent mRNA decay. This process is initiated by deadenylation of the mRNA molecule by one of the deadenylase complexes recruited by GW182. GW182 interacts directly with two deadenylase complexes, CCR4-NOT and, to a lesser extent, PAN2-PAN3 (Braun et al. 2011). The deadenylase complexes produce an unstable mRNA molecule without a poly(A)-tail. Subsequently, the miRISC proteins initiate removal of the 5′-cap (m7G) of the target mRNA. This process is called decapping and requires activators mRNA-decapping enzyme 1 (DCP1), Me31B, and HPat, which are recruited by the miRISC. These factors attract mRNA-decapping enzyme 2 (DCP2), which catalyzes decapping of the target mRNA (Nishihara et al. 2013). This is an irreversible process that commits the mRNA to full degradation by the major cytoplasmic 5′–3′ exonuclease XRN1 (Braun et al. 2012).
Studies on the relationship between translational repression and mRNA decay have demonstrated that translational repression contributed little to the repression of endogenous mRNAs in comparison with mRNA decay (10–25 % and 66–90 %, respectively) (Eichhorn et al. 2014; Guo et al. 2010; Hendrickson et al. 2009). However, these global measurements of translational efficiency and mRNA levels were often made at relatively late time points after introducing miRNAs. Therefore, they are thought to reflect the long-term steady-state effects of miRNAs. Two recent studies examined the initial effect of miRNAs on inducible reporter genes. They concluded that miRNAs predominantly exert their effects through translational repression on newly synthesized targets. This step is followed by mRNA deadenylation, decapping and subsequent mRNA decay, which is the dominant effect of miRNAs at steady state conditions (Djuranovic et al. 2012; Béthune et al. 2012).
Abovementioned mechanism enables miRNAs to regulate gene expression at a post-transcriptional level. As earlier mentioned, miRNAs are differentially expressed in different cells and cell types, including embryonic stem cells (ESCs) , induced pluripotent stem (iPS ) cells , and fully differentiated cells, resulting in different gene expression profiles and cellular properties (Wilson et al. 2009; Houbaviy et al. 2003). Many studies have examined genome-wide miRNA expression profiles of stem cells during differentiation or self-renewal to improve understanding of miRNAs involved in the regulatory networks of these cellular processes (Mallon et al. 2014; Razak et al. 2013).
6.2 The Role of microRNAs in Pluripotent Stem Cells
6.2.1 Introduction
Stem cells are undifferentiated cells that are able renew themselves, and differentiate into specialized cells to regenerate tissues . The potency of stem cells describes the potential to differentiate into different cell types. Totipotent stem cells can differentiate into every embryonic and extra embryonic cell type, and are able to generate an entire organism. However, only cells produced by the first few divisions of a fertilized egg are totipotent. Pluripotent cells are descendants of totipotent cells and can differentiate into cells of all embryonic cell types, but not extra embryonic cell types. Furthermore, adult multipotent stem cells, often termed progenitor cells, can only differentiate into several closely related cell types (Mitalipov and Wolf 2009). Pluripotent stem cells hold significant potential for clinical therapies because they theoretically possess the capacity to regenerate all types of tissue. However, these characteristics are only displayed by cells of the inner cell mass (ICM) from early embryos. Fortunately, early studies demonstrated that the pluripotent state of stem cells can be captured by placing pre-implantation blastocysts in culture, leading to the generation of pluripotent embryonic stem cells (ESCs) (Evans and Kaufman 1981).
Furthermore, the derivation of complete adult and sexually mature animals by transplantation of somatic nuclei into enucleated oöcytes demonstrated that somatic nuclei also withhold the information to generate a complete adult organism (Gurdon et al. 1958). Further studies demonstrated that differentiated cells can also be reprogrammed to pluripotent stem cells by nuclear transfer or cell fusion (Campbell et al. 1996; Blau et al. 1983). A real breakthrough in the reprogramming of somatic cells was made when Shinya Yamanaka invented the induced pluripotent stem (iPS ) cell technology in 2006. Yamanaka and co-workers demonstrated that pluripotency can be induced by introducing four transcription factors, Oct4, Sox2, Klf4, and c-Myc (OSKM), in mouse adult fibroblasts by retroviral transfection (Takahashi and Yamanaka 2006). This approach offers the opportunity to generate pluripotent stem cells from differentiated cells of an individual, which opened the field of personalized regenerative medicine. Unfortunately, reprogramming with OSKM has remained slow (2–4 weeks) and inefficient (0.01–0.1 % of total cells) (Stadtfeld and Hochedlinger 2010).
In the last few years, a variety of pluripotent and adult stem cells have been proposed to promote cardiac regeneration . The heart itself possesses little regenerative capacity, since cardiomyocytes exhibit a very slow turnover and the heart lacks a sufficient stem cell reservoir to regenerate itself. In this regard, pluripotent stem cells and progenitor cells have been proposed to replenish the loss of cardiomyocytes or to induce proliferation and differentiation of resident stem cells in the heart. Several subsets of stem cells and cardiac progenitor cells have shown experimental and clinical benefits. Alas, the effects of cell-based therapy have been modest, often due to low engraftment of applied cells and the limited differentiation into cardiovascular cell types of adult progenitor cells (Li et al. 2012; Balsam et al. 2004). In contrast, ESCs and iPS cells are able to differentiate into all cardiovascular lineages. However, efficient differentiation before transplantation is necessary since pluripotent cells may lead to teratomas after delivery in patients (Sun et al. 2010).
Because miRNAs are powerful regulators that control gene expression networks, they might represent useful tools to promote reprogramming of somatic cells into iPS cells or direct somatic cells to specific lineages in vivo and in vitro . Understanding miRNAs involved in self-renewal, reprogramming and differentiation may enable the development of safer and more efficient cell therapies or lead to the development of in vivo reprogramming strategies to regenerate the human heart.
6.2.2 microRNAs in Regulation of Pluripotency
The importance of miRNAs in the regulation of self-renewal and pluripotency came to light in studies with Dicer- and DGCR8-deficient mouse ESCs (mESCs) (Wang et al. 2007; Murchison et al. 2005) that lacked canonical miRNA processing. Disruption of both Dicer alleles resulted in embryonic lethality, indicating the importance of miRNAs in development. However, it is possible to generate proliferating Dicer-deficient mES cell lines, which indicates that canonical miRNA processing is not required for the maintenance of self-renewal. Nevertheless, Dicer-deficient mESCs do show severe defects in cell cycle progression and differentiation . This might be expected, since almost 50 % of all present miRNA molecules in mESCs, are produced from four loci (miR-21, miR-17–92 cluster, miR-15b-16 cluster and miR-290 cluster) involved in cell cycle regulation and oncogenesis (Calabrese et al. 2007). Similar to Dicer disruption, deletion of both DGCR8 alleles resulted in mouse embryonic lethality early in development, again indicating the importance of canonical miRNA processing in development. Furthermore, DGCR8-deficient mES cell lines also displayed defects in differentiation and cell cycle progression (Wang et al. 2007). In general, loss of miRNAs in mESCs compromises the exit of self-renewal and progression of the cell cycle.
Further studies have focussed on miRNA profiling of mESCs and human ESCs (hESCs ), which have revealed specific miRNA expression profiles. Interestingly, only a limited number of miRNAs are transcribed in a pluripotent stem cell state, and these are immediately silenced once cells receive differentiation signals (Houbaviy et al. 2003). Furthermore, reprogramming factors Oct4, Sox2, Nanog and c-Myc, which are able to induce pluripotency in somatic cells, occupy promoters of these miRNA families, hereby regulating their expression (Marson et al. 2008). The most abundant and ESC-specific miRNAs are transcribed from two miRNA clusters: the miR-290 (human homologues miR-371–373) cluster and the miR-302–367 cluster .
Transcription of miR-302–367 is regulated by Oct4 and Sox2 in hESCs and mESCs (Marson et al. 2008; Card et al. 2008). This miRNA cluster encodes miR-302a, miR302b, miR302c, miR-302d, and miR-367 (Suh et al. 2004), and promotes pluripotency by targeting several pathways. Lipchina et al. (2011) identified a list of 146 validated miR-302–367 targets through complementary experimental and computational approaches (Lipchina et al. 2011). The miR-302–367 cluster members silence inhibitors of cell survival and G1-S transition of the cell cycle. Repression of these targets contributes to the unusual short G1 cell cycle of ESCs, necessary to maintain rapid self-renewal of pluripotent stem cells (Wang et al. 2008a). Furthermore, miR-302–367 targets genes that promote heterochromatin formation to ensure an open chromatin formation, which is characteristic for pluripotent cells. Other targets of miR-302–367 include genes involved in metabolic regulation to promote glycolytic metabolism. By downregulating genes involved in oxidative phosphorylation and mitochondrial biogenesis, miR-302–367 promotes a metabolic state characteristic for ESCs (Lipchina et al. 2012). Another set of target genes of miR-302–367 is involved in intracellular vesicle transport and endocytosis. Although the exact role of intracellular vesicles and endocytosis in maintenance of self-renewal and pluripotency is unclear, studies have indicated that these processes play a role in proliferation (Szczyrba et al. 2011) and signalling pathways involved in development (Weigert et al. 2004).
The miR-290 cluster comprises the most highly expressed miRNAs in mESCs (>70 %) and possesses similar or identical seed sequences as the miR-302–367 cluster (Houbaviy et al. 2003). The human genome contains homologues of the miR-290 cluster, namely the miR-371–373 family (Wang et al. 2013). Besides the miR-302–367 cluster, certain members of the miR-290 cluster (miR-291a, miR-294, and miR-295) also regulate the G1-S phase transition during the cell cycle by primarily targeting cyclin-Cdk inhibitors (Wang et al. 2008a). Furthermore, Oct4 physically occupies the promoter of the miR-290 cluster, regulating its expression (Marson et al. 2008). MiR-290 targets include epigenetic repressive DNA methyltransferases, such as retinoblastoma-like protein 2 (Rbl2 ) (Sinkkonen et al. 2008). By downregulating Rbl2, this miRNA cluster promotes expression of Oct4, which in return promotes expression of the miR-290 cluster. Other targets of miR-290 include the NF-κB subunit p65, which normally promotes differentiation of ESCs (Lüningschrör et al. 2012). According to a recent study, the cluster also promotes glycolytic ESC metabolism by targeting Mbd2, which normally represses glycolytic metabolism (Cao et al. 2015). Though the miR-290 cluster is not expressed in hESCs, the homologous miR-371–373 family is predicted to target the same pathways in hESCs since almost all members from both clusters share the same seed sequence.
Interestingly, the seed sequence (AAGUGCU) of members of miR-290 and miR-302–367 clusters is shared by other miRNAs highly expressed in ESCs, including members of the miR-17–92 cluster, miR-106a–363 cluster, and miR-106b–25 cluster (Fig. 6.2) (Li and He 2012). Conservation of seed sequences between miRNA clusters expressed in ESCs indicates common mechanistic roles and partial redundancy of miRNAs in maintenance of self-renewal and pluripotency. However, these clusters also contain members with different seed sequences that might attribute to distinct characteristics of pluripotent stem cells of different species (Table 6.1) (Nichols and Smith 2009).
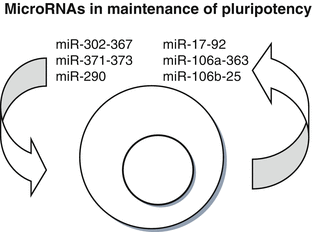
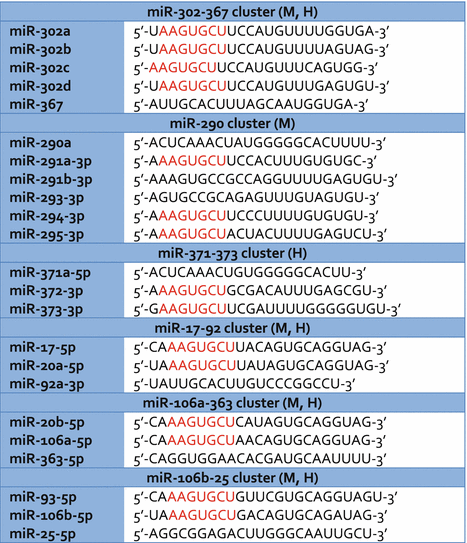
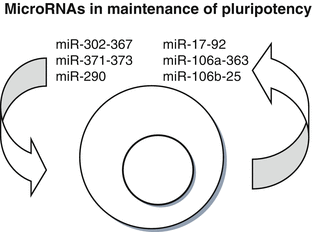
Fig. 6.2
MicroRNAs in the maintenance of pluripotency. The figure displays miRNA clusters that are involved in the maintenance of self-renewal and pluripotency. These miRNA clusters posses several members with similar or identical seed sequences (AAGUGCU), indicating common mechanistic roles of miRNAs in these processes
Table 6.1
MiRNA clusters expressed in mESCs and hESCs
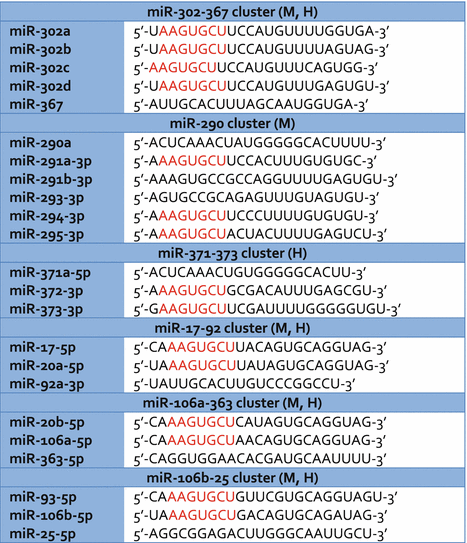
6.2.3 microRNAs in Alternate States of Pluripotency
Pluripotent stem cells of different species exhibit different characteristics and cellular responses. The ‘naïve’ pluripotent stem cell state is only represented by mESCs derived from the ICM of murine blastocysts. This state is characterized by expression of Oct4 by a distal enhancer, global reduction of DNA methylation, and repressive chromatin marks on developmental regulatory gene promoters (Marks et al. 2012). In contrast, hESCs are termed ‘primed’ pluripotent cells, and display more similarity to cells derived from the post-implantation murine embryonic epiblast with regard to gene expression patterns, epigenetic state, and signalling response to maintain an undifferentiated state (Tesar et al. 2007). These epiblast cells are called epiblast stem cells (EpiSCs ) and are thought to represent a less pluripotent state than ‘naïve’ pluripotent cells, since they cannot contribute to blastocyst chimeras (Tesar et al. 2007). Properties of ‘naïve’ and ‘primed’ pluripotent stem cells are elaborately reviewed elsewhere (Nichols and Smith 2009).
A limited number of studies have investigated differences in miRNA expression profiles between alternate states of pluripotency. Nevertheless, these studies have demonstrated that mESCs predominantly express the miR-290 cluster, preceding miR-302–367 expression at the early epiblast stage later in development (Spruce et al. 2010). EpiSCs and hESCs predominantly express the miR-302–367 cluster (Kim et al. 2011). These findings indicate that miR-302–367 expression corresponds with a slightly less pluripotent cell state.
Several studies have suggested that human pluripotent stem cell lines (hESCs and human iPS cells) exhibit different developmental potential, which translates into varying differentiation propensity (Osafune et al. 2008), especially concerning neural cell types (Hu et al. 2010) or hemangioblastic lineages (Feng et al. 2010). Differentiation propensity is very important to consider when using cell sources for cardiac regeneration. In a study by Kim et al. (2011), hESCs and iPS cells that expressed high levels of the miR-371–373 cluster demonstrated higher level of ‘naïve’ ESC markers and greater neural differentiation propensity. Their results suggest that human pluripotent cell lines that highly express the miR-371–373 cluster may represent a more ‘naïve’ pluripotent state, corresponding with greater differentiation propensity (Kim et al. 2011).
Due to their high pluripotent state, self-renewal, and ability to differentiate into all cells of the cardiovascular lineage, ESCs and iPS cells appear to be the ideal cell source for cardiac regeneration. However, clinical use of pluripotent stem cells is hampered by significant obstacles, including safety concerns, immune rejection, and low efficiency of iPS cell generation (Nussbaum et al. 2007; Okano et al. 2013). Since miRNAs perform an important regulatory function in self-renewal and pluripotency, they may improve reprogramming efficiency or function as a strategy for iPS cell generation without the use of viral vectors.
6.2.4 microRNAs in Reprogramming
Transduction of fibroblasts with transcription factors OSKM directly affects expression of several miRNA clusters, including miR-290 (and human homolog miR-371–373) (Judson et al. 2009; Subramanyam et al. 2011), miR-302–367 (Subramanyam et al. 2011), and paralogous clusters miR-17–92, miR106b–25, and miR106a–363 (Li et al. 2011). Since c-Myc and Klf4 are involved in oncogenesis, they are best avoided during iPS cell generation when considering clinical applications (Dang 2012; Yu et al. 2011). Therefore, researchers have attempted to generate iPS cells and enhance reprogramming efficiency using aforementioned miRNAs in combination with reduced numbers of pluripotency factors.
Based on the high expression levels of the miR-290 cluster in mESCs, first attempts of miRNA-mediated reprogramming involved overexpression of different members of this cluster in mouse embryonic fibroblasts (Wang et al. 2008a). Addition of miR-291-3p, miR-294, and miR-295 increased the efficiency of reprogramming in combination with retroviral transfection of Oct4, Sox2, and Klf4 (OSK) (Judson et al. 2009). miR-294 demonstrated greatest increased efficiency of up to 75 % of that achieved with OSKM (Judson et al. 2009). Therefore, it was suggested that miR-294 can efficiently substitute for c-Myc when reprogramming fibroblasts into iPS cells .
Furthermore, members of the miR-302–367 cluster that share the same seed sequence with members of the miR-290 cluster, also demonstrated the ability to improve reprogramming efficiency (Judson et al. 2009). Recent studies suggest that miR-302 improves reprogramming efficiency by suppressing several targets, including a repressor of Oct3/4 (NR2F2) (Hu et al. 2013) and several epigenetic regulators (AOF2, AOF1, MECP1-p66, and MECP2) to promote Oct3/4 expression and open chromatin formation, respectively (Lin et al. 2011).
Three other miRNA clusters (miR-17–92 cluster, miR-106b–25 cluster, and the miR-106a–363 cluster), were shown to be highly expressed during early stages of reprogramming with OSKM (Li et al. 2011). Overexpression of miR-106b–25 members, miR-93 and miR-106b, increased reprogramming efficiency fourfold in fibroblasts transfected with OSK. Li et al. (2011) have shown that miR-93 and miR-106b target the TGFβ-receptor 2 (TGFbr2) and cyclin-Cdk inhibitor p21 (Li et al. 2011). TGFbr2 is a receptor kinase that induces epithelial-to-mesenchymal transition (EMT) upon TGF-β signalling, a process were epithelial cells lose their cell-cell contacts and gain migratory and invasive properties (Thiery et al. 2009). Fibroblasts , a product of EMT, can only be reverted to a pluripotent state by repressing pro-EMT signalling (i.e. TGF-β signalling), hereby initiating the reverse process, mesenchymal-to-epithelial transition (MET) (Li et al. 2010). Consistent to these findings, embryonic stem cells are morphologically similar to epithelial cells and express E-cadherin, an epithelial marker (Baum et al. 2008). Moreover, human miR-302b and human miR-372 were also found to inhibit TGFβ-signalling by targeting TGFbr2. Addition of these miRNAs to OSK or OSKM resulted in a 10- to 15-fold increase of reprogramming efficiency, respectively (Subramanyam et al. 2011).
In addition to miR-290, miR-302–367, miR-17–92, and miR-106b–25 cluster members, a recent library screen of 379 miRNAs observed improved reprogramming efficiency during reprogramming with OSK in combination with the miR-130–301–721 family (Pfaff et al. 2011). This newly identified miR-family is indirectly involved in cell cycle regulation by targeting transcription factor Meox2, which normally promotes expression of cell cycle inhibitor p21 (Pfaff et al. 2011).
Instead of resembling miRNA expression profiles of pluripotent cells, an alternative approach to promote reprogramming with miRNAs might be to reduce expression of miRNAs that are highly expressed in differentiated fibroblasts. Let-7 family miRNAs, which are specifically depleted in ESCs, repress pluripotency factors and are highly expressed during differentiation. Interestingly, let-7 and miR-290 cluster miRNAs perform opposite functions in ESC self-renewal and pluripotency (Melton et al. 2010). It has been demonstrated that Let-7 members directly target several ESC-specific genes , including c-Myc, and downregulate important cell cycle molecules such as Cdk4 and CyclinD , to repress the G1-S transition of the cell cycle (Melton et al. 2010; Schultz et al. 2008). Accordingly, let-7 miRNA inhibition resulted in a fourfold increase of reprogramming efficiency compared to three pluripotency factors (OSK) alone (Melton et al. 2010). Repression of tumor suppressor pathways also appears important during the generation of pluripotent cells. For instance, depletion of miR-34 (Choi et al. 2011) and inhibition of miR-199a-3p (Wang et al. 2012), which act downstream of tumor suppressor p53, enhanced OSKM reprogramming efficiency. Moreover, by directly targeting p53 mRNA, ectopic expression of mir-138 (Ye et al. 2012) also significantly improved the efficiency of iPS cell generation. However, p53 is shown to preserve genomic integrity by aborting reprogramming in cells carrying various types of DNA damage, normally resulting in p53-dependent apoptosis (Marión et al. 2009). This reserves a crucial role for p53 in preventing iPS cell generation from suboptimal cells (Marión et al. 2009). Therefore, p53-related miRNAs have to be further investigated before they can be safely used to enhance reprogramming. To date, repression of miRNAs specific for differentiated cells has only slightly increased the efficiency of reprogramming without transcription factor c-Myc (Yang et al. 2011).
Besides stimulating or repressing miRNAs to promote reprogramming efficiency, miRNAs have also been investigated to induce reprogramming without the addition of transcription factors. Only a few studies have reported miRNA-mediated reprogramming of somatic cells to iPS cells without additional reprogramming factors. Reprogramming in the absence of transcription factors was first achieved by expression of the miR-302–367 cluster in cancer cells (Lin et al. 2008). Further investigations of Lin et al. led to the generation of iPS cells through inducible miR-302s (a viral vector containing miR-302a, b, c and d) expression (Lin et al. 2011). Recently, Anokye-Danso et al. (2011) reported that overexpression of miR-302–367 cluster members successfully reprogrammed mouse and human fibroblasts into iPS cells in the absence of additional factors (Anokye-Danso et al. 2011). Direct transfection of mature double-stranded miR-200c in combination with miR-302 and miR-369 was also reported to be sufficient to induce pluripotency in somatic cells (Miyoshi et al. 2011). Lüningschrör et al. (2013) failed to reproduce these miRNA-mediated reprogramming experiments and could not induce pluripotency with miRNAs alone (Lüningschrör et al. 2013).
In summary, it has been demonstrated that miRNAs that target cell cycle inhibitors, epigenetic regulators, and modulators of mesenchymal-to-epithelial transition, clearly enhance reprogramming efficiency of somatic cells into iPS cells. Additional investigations are necessary to reveal if miRNAs alone can be used to effectively reprogram fibroblasts to iPS cells without the use of reprogramming factors (Fig. 6.3).
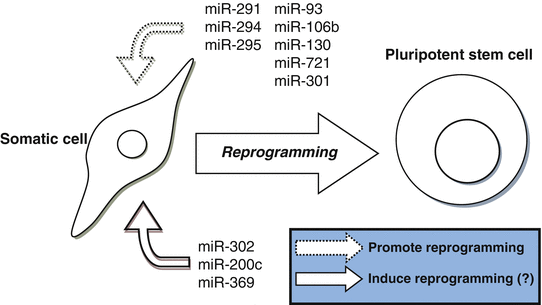
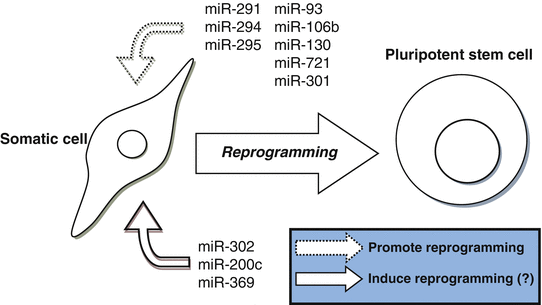
Fig. 6.3
MicroRNAs involved in reprogramming. The figure displays miRNAs that have demonstrated to promote reprogramming efficiency of somatic cells into iPS cells or were reported to induce reprogramming into iPS cells without additional transcription factors. However, the ability of miRNAs to reprogram cells without additional factors remains questionable
6.3 The Role of microRNAs in Cardiovascular Lineage Commitment
6.3.1 Introduction
Considering safety concerns with regard to pluripotent stem cells, differentiation of iPS cells and ESCs into specific lineages before transplantation is necessary. Canonical miRNAs play an essential role in cardiac development, as studies have shown that cardiac-specific knockout of the miRNA-processing enzyme Dicer results in rapid dilated cardiomyopathy, heart failure, and embryonic lethality (Chen et al. 2008). Since miRNAs are powerful regulators of gene expression patterns, they might represent an efficient strategy to modulate commitment of cardiac progenitor cells (CPCs) and pluripotent stem cells to cardiovascular lineages ex vivo or in vivo (summarised in Table 6.2).
Table 6.2
MiRNAs in cardiovascular lineage commitment
Endothelial cells ![]() | Cardiomyocytes ![]() | Vascular smooth muscle cells ![]() | |||
---|---|---|---|---|---|
miR-126 | Essential for vascular integrity and angiogenesis | miR-1 | Modulates proliferation and promotes cardiomyocyte differentiation | miR-1 | Required for VSMC differentiation from ESCs |
Not sufficient to induce EC differentiation | miR-133 | Represses smooth muscle genes | miR-10a | Inhibition blocks VSMC differentiation from ESCs | |
miR-17–92 | Inhibition of miR-92a enhances neo-vascularisation | Overexpression inhibits cardiomyocyte differentiation | |||
Blocks ischemia-induced angiogenesis, enhances tumour-associated angiogenesis | miR-208a | Regulates cardiomyocyte hypertrophy | miR-143/145 | Promotes VSMC differentiation | |
miR-99b, miR-181a/b | Promote EC differentiation from ESCs | miR-499 | Increases cardiomyocyte differentiation | Necessary to acquire and maintain contractile phenotype (maturation) | |
miR-130, miR-210 etc. | Several miRNAs promote angiogenesis and are upregulated during EC differentiation | miR-221/222 | Promote VSMC proliferation by targeting cyclin-cdk inhibitors |
6.3.2 microRNAs in Cardiomyocyte Differentiation and Proliferation
Specific miRNAs involved in cardiac differentiation have been revealed by miRNA expression profiling of hESC-derived cardiomyocytes and during differentiation of CPCs, which highlighted changes in several miRNAs. Especially expression of miR-1, miR-133, miR-208, and miR-499, changed remarkably during cardiac differentiation (Sluijter et al. 2010; Ivey et al. 2008; Wilson et al. 2010).
miR-1 and miR-133 are highly expressed in cardiac and skeletal muscle cells, where they modulate muscle proliferation and differentiation while repressing other lineages (Ivey et al. 2008; Chen et al. 2006). In humans, miR-1 and miR-133 are under regulation of cardiac and muscle specific transcription factors, including serum response factor (SRF ), myocyte enhancer factor 2 (MEF2 ), and myoblast determination protein (MyoD ) (Liu et al. 2007; Zhao et al. 2005). Although miR-1 and miR-133 are transcribed together as a polycystronic cluster, they appear to perform distinct functions during proliferation and differentiation of myoblasts (Chen et al. 2006). miR-1 promotes myogenesis by targeting histone deacetylase 4 (HDAC4), a transcriptional repressor of myogenic gene expression. Furthermore, by directly targeting frizzled family receptor 7 (FZD7) and fibroblast growth factor receptor substrate 2 (FRS2), miR-1 suppresses WNT and FGF signalling, which promotes cardiomyocyte differentiation (Lu et al. 2013). By targeting Hand2, a transcription factor required for ventricular cardiomyocyte proliferation, miR-1 also modulates the growth of the developing heart. Accordingly, miR-1 deficient mice displayed enhanced Hand2 expression, which resulted in an abnormally enlarged heart (Zhao et al. 2007). Consistently, miR-1 overexpression resulted in thin-walled ventricles of the heart in a mouse model, indicating premature cardiomyocytes due to early exit of the cell cycle and hampered cardiomyocyte proliferation (Zhao et al. 2005). Furthermore, miR-1 overexpression promotes cardiomyocyte differentiation from cardiac progenitor cells and ESCs of both mouse and human origin, demonstrated by an increase in cardiac marker expression (Sluijter et al. 2010; Lu et al. 2013).
In contrast to miR-1, which promotes differentiation, overexpression of miR-133 represses cardiac differentiation of hESCs and mESCs (Ivey et al. 2008). Furthermore, miR-133 directly targets muscle transcription factor SRF, suppressing smooth muscle gene expression (Liu et al. 2008). Accordingly, complete deletion of both mir-133 alleles results in ectopic activation of smooth muscle genes in the developing heart, as well as excessive proliferation and apoptosis of cardiomyocytes (Liu et al. 2008). Moreover, miR-133 overexpression in mouse models led to ventricular wall thinning and decreased cardiomyocyte proliferation (Liu et al. 2008; Carè et al. 2007). Altogether, studies have shown that polycystronic transcription of miR-1/miR-133 results in adequate regulation of cardiac proliferation and cardiomyocyte differentiation during cardiac development.
Further studies on miRNAs in cardiac development identified a family of intronic miRNAs, termed ‘myomiRs’, consisting of miR-499, miR-208a and miR-208b, which share many target genes. Although miR-208b and miR-499 are expressed in skeletal and cardiac muscle, miR-208a is only expressed in the heart where it regulates cardiomyocyte hypertrophy (Liu and Olson 2010; Callis et al. 2009). miR-499 is highly expressed in cardiac progenitor cells (Sluijter et al. 2010) and the heart (van Rooij et al. 2009), and in hESCs during cardiac differentiation (Wilson et al. 2010). Overexpression of miR-499 in human cardiac progenitor cells reduced proliferation of these cells and induced differentiation into cardiomyocytes (Sluijter et al. 2010). Furthermore, injection of miR-499 into the border zone of infracted rat hearts enhanced cardiomyogenesis in vivo (Sluijter et al. 2010). Consistent with these findings, expression of miR-499 resulted in upregulation of cardiac markers, such as Nkx2.5 and GATA4, by repression of SOX6 and PTBP3. In contrast, inhibition of miR-499 in mouse and human ESCs inhibits cardiomyocyte differentiation (Sluijter et al. 2010). In the mammalian heart, miR-499 is expressed at very low levels in c-kit positive cardiac stem cells, while it is highly expressed in cardiomyocytes, together with miR-1 and mir-133 (Hosoda et al. 2011). This suggests that these miRNAs are important in the specialization of cells towards a cardiomyocyte cell fate. Interestingly, miR-499 was demonstrated to translocate through gap junctions from cardiomyocytes to c-kit positive cardiac stem cells (CSCs) to promote differentiation into de novo cardiomyocytes (Hosoda 2013).
An alternative strategy to regenerate the heart is to stimulate endogenous cardiomyocyte proliferation. Several studies have shown that zebrafish are able to generate new myocardium from existing cardiomyocytes (Kikuchi et al. 2010; Jopling et al. 2010). During zebrafish heart regeneration, cardiomyocytes around the cardiac injury dedifferentiate into a more immature phenotype and cardiac transcription factors, such as Gata4 and Hand2, are activated (Kikuchi et al. 2010; Schindler et al. 2014). Subsequently, these dedifferentiated cardiomyocytes re-enter the cell cycle and fully repair the injured heart (Jopling et al. 2010). Decreased expression of miR-133 was observed by Yin et al. (2012) during zebrafish heart generation. Accordingly, miR-133 depletion enhanced cardiomyocyte proliferation by elevating repression of cell cycle factors and cell junction components (Yin et al. 2012). More recently, Aguirre et al. (2014) identified two miRNA families, miR-99/100 and let-7a/c, which were also downregulated during zebrafish heart regeneration. Alas, miR-99/100 expression remained unchanged after cardiac injury in mice. Nevertheless, knockdown of miR-99/100 and let-7a/c in mice reduced scar size and improved cardiac function after myocardial injury (Aguirre et al. 2014). Recently, direct injection of viral vectors expressing human miR-590 and miR-199a promoted cardiomyocyte proliferation and restored cardiac function in mice by targeting genes involved in cell cycle and proliferation (Eulalio et al. 2012). Altogether, these results indicate that regenerative mechanisms of the heart may be conserved between species, although they are not sufficiently stimulated upon cardiac injury in mammals.
6.3.3 microRNAs in Vascular Smooth Muscle Differentiation
The importance of miRNAs in vascular smooth muscle cell (VSMC) development was revealed during experiments with mutant mice that possessed a dysfunctional Dicer allele under a VSMC-specific promoter. Mutant mice died at embryonic day 16 or 17 due to bleeding and impaired VSMC contractility. Interestingly, these vascular defects observed in mutant mice could be partially rescued by overexpression of miR-145, highlighting the importance of this specific miRNA in VSMC development (Albinsson et al. 2010).
miR-145 is the most highly expressed miRNA in VSMCs, and is transcribed together with miR-143. During development, miR-145/143 is expressed in VSMCs under control of Nkx2.5, SRF, and its co-activator, myocardin. These miRNAs target a number of transcription factors, including Klf4 and Elk1 (both involved cellular proliferation), as well as a number of cytoskeletal proteins, to promote differentiation and repress proliferation of VSMCs (Cordes et al. 2009). In addition, miR-145, but not miR-143, is necessary for myocardin-induced reprogramming of fibroblasts into VSMCs and overexpression of miR-145 induced VSMC differentiation from neural crest cells (Cordes et al. 2009). Inhibition of miR-145 did not fully inhibit VSMC differentiation from ESCs but resulted in a more immature SMC phenotype (Cordes et al. 2009). Furthermore, miR-143/145 expression appears necessary for VSMCs to acquire and maintain a contractile phenotype (Boettger et al. 2009). Loss of these miRNAs results in impeded neointima formation because miR-143/145 act downstream of SRF during cytoskeletal remodelling and VSMC migration after vascular injury (Xin et al. 2009). Surprisingly, overexpression of miR-143 and miR-145 also decreased neointima formation in a rat model of acute vascular injury (Elia et al. 2009). These data indicate that miR-143 and miR-145 play crucial roles in modulating VSMC maturation and migration, though they are not essential for VSMC differentiation from stem cells.
Further studies have identified miR-1 , which also mediates cardiomyocyte differentiation, as one of the regulators in VSMC differentiation (Xie et al. 2011). miR-1 is required for VSMC differentiation from ESCs, as inhibition of miR-1 led to a down-regulation of VSMC-specific markers and decreased the number of VSMCs from ESCs. Similar to miR-145, miR-1 also targets transcription factor Klf4 (Xie et al. 2011). In addition to miR-1, miRNA profiling during VSMC differentiation demonstrated an increased expression of miR-10a, which was shown to target HDAC4 (Huang et al. 2010). Accordingly, inhibition of miR-10a blocked VSMC differentiation from ESCs. However, it has not been revealed how repression of HDAC4 by miR-10a is specifically involved in VSMC differentiation. Furthermore, miR-221 and miR-222 were found to be involved in VSMC proliferation by targeting cyclin-dependent kinase inhibitors, p57 and p27 (Davis et al. 2009; Liu et al. 2009). Consistent with these findings, knockdown of miR-221 and miR-222 suppressed VSMC proliferation in vivo (Liu et al. 2009). More recent studies on VSMC differentiation and proliferation have identified other miRNAs that either promote or inhibit VSMC differentiation. miRNAs that promote differentiation include miR-663 (Li et al. 2013) and miR-18a-5p (Kee et al. 2014), while others such as miR-132 (Choe et al. 2013), and miR-365 (Zhang et al. 2014) inhibit VSMC differentiation. Further studies are necessary to discover the potential of these miRNAs with regard to heart regeneration after injury.
6.3.4 microRNAs in Endothelial Differentiation and Vascular Development
Early studies demonstrated that Dicer-deficient mouse embryos exhibited defects in vasculogenesis and angiogenesis, which was consistent with altered expression of vascular marker genes such as vascular endothelial growth factor (VEGF), vascular endothelial growth factor receptor-1 and receptor-2, and Tie1 (Yang et al. 2005). Later in development, these Dicer-deficient mice had an impaired angiogenic potential after vascular injury (Suárez et al. 2008).
The most extensively studied angiogenic miRNA is miR-126, which was demonstrated to be highly important for vessel integrity and angiogenic signalling by early studies in zebrafish and mice (Fish et al. 2008; Wang et al. 2008b). Targeted deletion of miR-126 resulted in a reduced angiogenic response to endothelial growth factors, loss of vascular integrity, and induced haemorrhages in zebrafish (Fish et al. 2008). In mice, loss of miR-126 also led to leaky blood vessels, emphasising the importance of miR-126 in vascular physiology. As identified by microarray analysis, loss of angiogenic potential and vascular integrity can, at least in part, be attributed to increased levels of Spred1 and Pik3r2, which are repressors of angiogenic signalling and direct targets of miR-126 (Fish et al. 2008; Wang et al. 2008b). Moreover, miR-126 has been demonstrated to modulate expression of stromal cell-derived factor 1 (SDF-1) in ECs, which is proposed to regulate recruitment of vascular progenitor cells to ischemic regions (van Solingen et al. 2011). In addition to pro-angiogenic effects, miR-126 regulates expression of inflammatory mediators in endothelial cells, such as vascular cell adhesion molecule 1 (VCAM-1), hereby limiting leukocyte adhesion and subsequent inflammation (Harris et al. 2008). Although enriched in endothelial progenitor cells (EPCs), miR-126 lacks the potential to induce endothelial cell differentiation in ESCs, as expression of several vascular marker genes was not affected by miR-126 overexpression (Fish et al. 2008). In summary, miR-126 is important for vessel integrity and angiogenesis by modulating angiogenic signalling, vascular progenitor cell recruitment, and inflammation. However, miR-126 is not sufficient to induce EC differentiation in ESCs, indicating a minor role in endothelial lineage commitment.
The miR-17–92 cluster, induced by transcription factor c-Myc, comprises of miR-17, -18a, -19a/b, -20a, and -92a, and is also expressed in ECs. Interestingly, this cluster has shown to perform distinct functions in different contexts. First, the miR-17–92 cluster plays an important role in angiogenesis after vascular injury (O’Donnell et al. 2005; He et al. 2005). One of the clusters members, miR-92a, performs anti-angiogenic functions in ECs by targeting several proangiogenic factors. Accordingly, inhibition of miR-92a enhanced neovascularisation and functional recovery after myocardial infarction (Bonauer et al. 2009). Furthermore, expression of miR-17, miR-18a, miR-19a, and miR-20a, has shown to inhibit angiogenic activity of ECs in vitro, while inhibition of these miRNAs led to stimulation of angiogenic processes (Doebele et al. 2010). In contrast, miR-17–92 cluster appears to enhance neovascularisation in tumour cells. Therefore, it is proposed that the effect of miR-17–92 cluster differs for ischemia-induced angiogenesis and tumour-associated angiogenesis, which is important to consider when developing clinical therapies (Dews et al. 2006).
< div class='tao-gold-member'>
Only gold members can continue reading. Log In or Register a > to continue
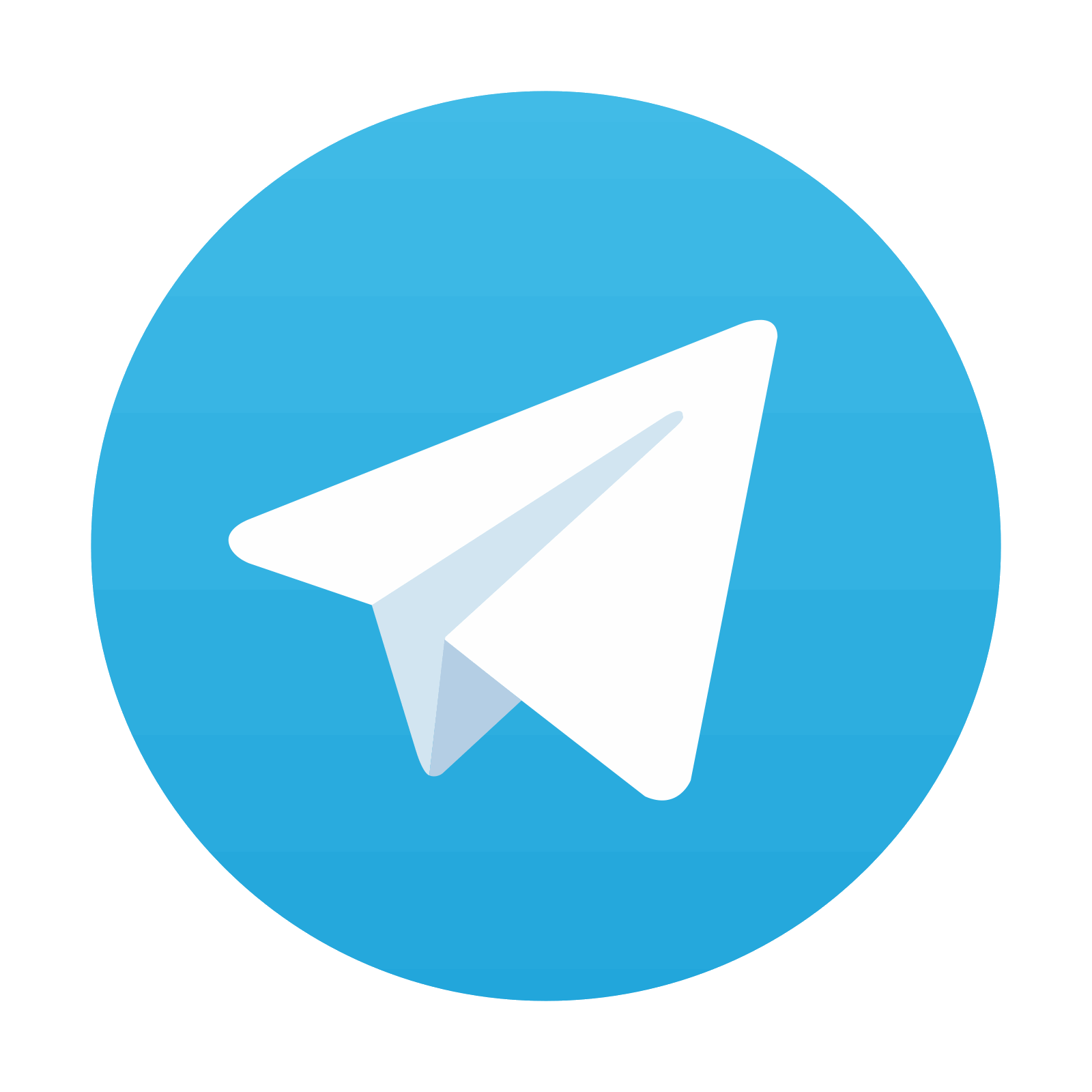
Stay updated, free articles. Join our Telegram channel
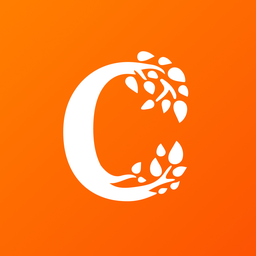
Full access? Get Clinical Tree
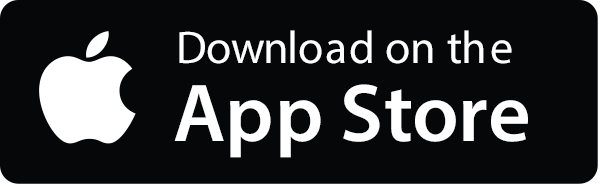
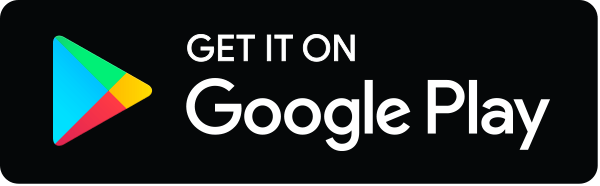