(1)
Kantonsspital Aarau, Medizinische Universitätsklinik, Aarau, Switzerland
Keywords
LV-afterloadPreloadTransmural LVEDPLV end-diastolic pressure (LVEDP)Stroke volumeLV end-diastolic volume (LVEDV)Pericardial pressure (PP)Central venous pressure (CVP)Cardiac output (CO)Fluid responsivenessFluid challengeExtravascular lung water (EVLW)AutoregulationTissue hypoperfusionLV contractilityFluid loading/volume expansionImpedance(Total) arterial/vascular complianceEffective arterial elastance (Ea)Effective ventricular elastanceLaw of LaPlaceAfterload mismatchSystemic vascular resistance (SVR)LV contractilityLV (RV) ejection fraction (EF)Ventriculo-arterial couplingInotropyDiastolic ventricular interaction (DVI)Pericardial constraintPulmonary hypertensionMyocardial stiffnessChamber (ventricular) stiffnessPressure-volume relation (PV-relation)RV end-diastolic pressure (RVEDP)RV end-diastolic volume (RVEDP)RV afterloadDiastolic ventricular propertiesVascular properties1.1 Cardiac Performance
Cardiac performance depends on a wide variety of factors, of which preload, afterload, heart rate, and contractility are the best recognised (Fig. 1.1). However, other factors play important roles but are less acknowledged. The diastolic ventricular interaction (DVI) and its impact on preload, the preload recruitable stroke-work, ventriculo-arterial coupling and other vascular and ventricular properties, through their interaction at end-systole, all have significant influence on cardiac performance.


Fig. 1.1
The modified diagram by Gould and Reddy, “Vasodilator Therapy for Cardiac Disorders”, Futura, Mount Kisco, New York, 1979, pp 1-6, illustrates the complex interplay of factors affecting cardiac performance. With permission
1.2 The Fundamental Equation of the Circulation

The fundamental equation of the circulatory system expresses the basic function of the heart: to generate flow and pressure in order to ensure appropriate perfusion of the body [3, 4].
The systemic peripheral resistance, difficult to determine directly in practice, can be calculated by using the measurable parameters of MAP and CO. However the SVR is not determined by them, SVR and CO are independent, the MAP is the dependent variable [5].
Poiseuille’s law offers three ways to change blood pressure [6, 7]:
alter flow,
alter resistance,
alter both.
Thus, increased blood flow and/or an increased ratio of resistance/blood flow (SVR/CO) can alter the MAP [8]. If CO and SVR change reciprocally and proportionately, only then will the MAP be unchanged. If CO increases but with a reduction of SVR due to peripheral vasodilatation, MAP will increase if the increase in CO is proportionately higher than the reduction of SVR. In the case of volume loading, increasing CO will lead to an increase in MAP if SVR remains unchanged [5].
Kumar showed that volume loading in healthy hearts increases contractility, stroke work, systolic blood pressure, and MAP [9]. However, in the heart with compromised contractility, blood pressure might not increase. Michard [10] showed that the increase in SV (flow) depends critically on the contractile abilities of the heart. Thus, if volume loading does not lead to an increase in SV, we should be suspicious of significant heart failure. Furthermore, we should keep in mind that, in heart failure syndromes, the LV afterload is the decisive determinant of cardiac performance [11–14]. Therefore, a reduction in afterload by vasodilators is the treatment of choice [15, 16].
As a rule, in daily clinical practice in acute heart failure when lowering peripheral resistance, the LV end-systolic wall stress will be reduced and the SV will increase, but the MAP will be maintained or will even increase [17–19]. If, under these conditions, the MAP does not increase or at least cannot be maintained, the following circumstances have to be considered:
1.3 Preload
1.3.1 Definition
Preload is defined by Braunwald and Ross [30] as “the force acting to stretch the left ventricular muscle fibres at the end of diastole and determining the resting length of the sarcomeres”.
Returning venous blood fills the ventricle, exerting force on the heart muscle, stretching the myofibrils [30] and is one of the main determinants of cardiac performance [31–33].
The end-diastolic ventricular volume, or preload, is well reflected by the end-diastolic wall stress (preload ~ end-diastolic wall stress) [34].
1.3.2 The Frank–Starling Mechanism
Transmural LVEDP accurately reflects the effective distending pressure responsible for the length of myocardial fibres [35].
Otto Frank [36] and Ernest Starling [37] obtained a relationship between the end-diastolic fibre length and the force of contraction:
With increasing fibre length the force of contraction increases and thus the LV or RV stroke volume (SV) [36, 37] increases or, more accurately, the stroke work (SW) increases:


The diastolic ventricular filling is limited by the acutely non-distensible pericardium constraining the filling ventricles and by the cytoskeleton [40–42], thus preventing the ventricles from fluid overload [43, 44] (physiological protective mechanism) as well as from pathological dilatation [41].
With an increase in resting fibre length the velocity of fibre muscle shortening increases as well [45].
Frank [36] established a linear relationship between the left ventricular end-diastolic volume (LVEDV) as a correlate of the fibre length and the force of ventricular contraction [30, 36, 37, 43].


[46]
Starling [37] reported an increase in the contraction force with increasing atrial pressures. Starling’s result is similar to that described by Frank, as long as the increase in LVEDP rep-resents a roportional increase in LVEDV (linear relationship between LVEDP and LVEDV).This is true in most healthy persons as long as the LVEDP remains within normal ranges, but in the case of high LV filling pressures and in certain pathological circumstances the rise in LVEDP is often disproportionately high in comparison to the increase in LVEDV [23, 24, 47–49].
The LVEDP may even rise without any increase in LV filling volume, producing no increase in preload, which is essential to recruit a higher SV [23, 39, 42]. Therefore, although the LVEDP rises, there may be no adequate increase in SV; in fact, there may even be a fall corresponding with the ‘descending limb’ of the Starling curve [35, 37, 39, 50]. This descending limb described by Starling is, however, an artefact of his experimental conditions.
When using the effective distending pressure rather than the intra-cavitary pressure the relation between fibre stretch and force of contraction is described adequately and corresponds to Frank’s findings and the statement:
The effective distending pressure or ‘transmural’ LVEDP is the intracavitary LVEDP (commonly just called LVEDP) minus the surrounding pressure(s) [35].
Katz, in 1965, already assumed that intracavitary and transmural end-diastolic left ventricular pressures were only equal when the pressure surrounding the left ventricular heart muscle was negligible [35]. Otherwise the external pressure must be subtracted from the intracavitary LVEDP to calculate the effective distending or transmural pressure.


[35]
Usually, the surrounding pressure has contributions of one-third by the RVEDP and two-thirds by the pericardial pressure [51, 52]:
Transmural LVEDP = intracavitary LVEDP − (2/3 pericardial pressure + 1/3 RVEDP) Under normal conditions, RAP and pericardial pressure (PP) are nearly equal [53–55] and further changes in pericardial pressure are very closely reflected by RA pressure changes [53, 56, 57].
The close relation between changes in RA pressure and pericardial pressures allows us to give a reasonable estimate of transmural pressure by subtracting RAP from pulmonary capillary wedge pressure (PCWP) [23, 53, 56]:


There is substantial evidence that PCWP reflects LVEDP [60–62]. CVP is measured where the vena cava leads into the right atrium [58] and, as such, equals the RAP [58, 59]. Due to the very close relations between RAP and PP (r = 0.95, p < 0.005) [63] and RAP and changes in PP [53, 56, 57] respectively, CVP is a good estimate of PP [53–55, 58, 59, 63] in daily practice. Furthermore, both, CVP and RAP reflect the RVEDP [44, 59, 63]. Over a wide range, pericardial pressure, RAP and RVEDP are literally equal [64]. Tyberg [53] demonstrated that RVEDP well represents PP in ranges between 4 and 20 mmHg. However, in case of right ventricular hypertrophy when the RV is stiffened [65] and in cor pulmonale or pulmonary hypertension, RAP and RVEDP are much higher than PP. RV-failure always cause a rise in CVP [ 58 ].
In healthy persons the surrounding pressure is low (nearly zero) and an increase in preload will increase the LVEDP more than the surrounding pressure [23, 41]. Hence, the transmural LVEDP will rise along with LVEDV [23, 43, 66], increasing the preload recruitable stroke volume (work) and thus SV, as described by Frank and Starling.
In conditions where the surrounding pressure rises substantially, external constraint increases more than LVEDP [23, 24, 47, 48, 67, 68]. Transmural LVEDP and intracavitary LVEDP will differ considerably and will change in opposite directions with a fall in transmural LVEDP, lowering the preload and, consequently, the preload recruitable stroke volume (work) will decrease.
The intraventricular pressures (intracavitary LVEDP and RVEDP) are influenced by:
The LV compliance describes the diastolic properties of the heart muscle and can be depicted by the relation between LVEDP and LVEDV [51, 72] (relation between pressure and volume).
With this in mind, the discrepancies between transmural LVEDP and intracavitary LVEDP can be related, at least partly, to the ventricular compliance [73].
The ventricular compliance varies almost continuously in the critically ill, producing changes in the intracavitary LVEDP but without any corresponding change in LVEDV [69, 74, 75]. Kumar [76], however, established evidence that continuous change in the ventricular compliance is a physiological phenomenon present in healthy persons as well as in those who are unwell.
In heart failure, the compliance of the LV is almost always reduced [50], hence, increases in filling volumes cause a higher rise in LVEDP compared to a healthy heart.
The compliance is determined by factors such as muscle mass, tissue composition, elastic properties, ventricular interactions and extramyocardial conditions including pericardial structure and intra-thoracic properties [77–80].
Raised intrathoracic pressure due to pneumonia, ARDS, pulmonary oedema, etc., as well as raised intra-abdominal pressure will increase constraint, in particular on the thin-walled RV, affecting the RVEDP and PP more than the LVEDP [47].
Furthermore, the higher the LVEDP the greater the amount of external force acting on the LV, thus impeding the LV-filling, the preload, and preload recruitable SV (SW) [24, 25].
Examples of situations which alter the surrounding pressures or produce significant external pericardial constraint are:
increased lung water due to congestive HF [81],
mechanical ventilation and PEEP: Both induce a rise in intrathoracic pressure (surroundingpressure) and an increase in RV-afterload [82]. The normally low RVEDP and PP will rise markedly in case of mechanical positive pressure ventilation and/or PEEP application, pneumonia, ARDS, etc., and so contribute essentially to an increase in the surrounding pressure [56];
In heart failure patients we expect a marked external constraint to be present in the majority of patients, compromising LV-filling and becoming significant if LVEDP > 10(12)–15 mmHg [24, 25, 83]. Physiological external constraint, mainly due to PP, contributes up to 30–40% of the LVEDP [25]. In heart failure the contribution to LVEDP by the external constraint is as high as 50–80% [23];
In the case of external constraint, LVEDP markedly overestimates effective distending pressure [42].
Changes in opposite directions (transmural LVEDP ↓ and intracavitary LVEDP ↑) are explainable now, and only an increase in transmural LVEDP is consistent with an increase in LVEDV and vice versa [23, 39, 42].
Numerous publications have established that haemodynamic monitoring by PA-catheterisation measuring intracavitary (filling-) pressures fails to be an accurate guide of the preload because filling pressures do not adequately reflect the myocardial fibre length at end-diastole and, hence, the LVEDV [36, 84–86]. If the transmural pressure is used instead, then changes in the preload are accurately reflected [35].
However, the filling pressures are still one of the most important components in assessment and treatment decision-making processes in heart failure. The heart always tries to generate an adequate CO on the lowest possible LVEDP [73, 86, 87]. In heart failure patients, a therapeutic reduction of the LVEDP is correlated with improved outcome [15, 88]; hence, unloading the left ventricle and reducing the LVEDP is the therapeutic maxim that adheres to the physiology/pathophysiology of the situation [23, 24, 36, 37, 50] and improves outcome [13, 15, 24, 88]. Thus, we might do much better in our patients with severe heart failure and cardiogenic shock using the transmural LVEDP to make our therapeutic decisions.
There is, of course, a physiological optimum and maximum of fibre distension and concomitant force development (see Fig. 1.2) [89].


Fig. 1.2
∆p: Change in pressure; ∆SV: Change in SV; with increasing transmural pressure and thus preload, the recruited SV becomes less (modified from Michard [10], with permission)
Furthermore, in the situation of (acute) heart failure the Frank–Starling mechanism is markedly diminished [50] and thus, in the failing heart, an increase in fibre stretch (ventricular filling) is not accompanied by the same increase in the force of contraction as in healthy persons [90].
1.3.3 Venous Return and CVP in Daily Practice
SV is determined by venous return (responsible for the preload) and cardiac performance (con- tractility, afterload and heart rate) [31–33].
Guyton et al. [32] evaluated the relationship between total cardiac function (contractility and total peripheral resistance) and venous return:
“The actual cardiac output changes with changes in cardiac function (CF), but with changes in venous return as well”.
Indeed, as increasing ventricular filling and (thus) ventricular stretch will lead to an increase in SV, is SV basically a function of end-diastolic filling volume [95], and as such (up to an upper limit—sarcomere length of 2.2 μm) in some aspects of the amount of venous return.
Guyton plotted the relationships (total cardiac function and venous return) on one graph (see Fig. 1.3) [32].


Fig. 1.3
The upper cardiac function curve depicts a supra-normal performance (i.e. ↑ sympathetic tone) while the lower curve represents the situation in H.F. Venous return: High – normal – low (adapted from Mohrman, DE and Heller, LJ Cardiovascular Physiology, 4th ed. McGraw-Hill Comp., 1997, chapter 9, p. 147), with permission
Under most physiological conditions, changes occur simultaneously in these relationships, although one effect will be dominant [31], for example:
If CO rises with a fall in right atrial pressure (central venous pressure), the dominant effect is improvement in cardiac function (increase in contractility and/or reduction in afterload);
If CO rises with an increase in RAP the dominant effect is an increase in volume, and a decrease in venous compliance or venous capacity, resulting in a higher venous flow for any pressure in the right atrium.
As such, the special interrelationship between CVP and CO has to be considered when interpreting hemodynamic conditions: Both, CVP and CO are determined by the interaction of the two functions, the cardiac function and the venous return [32, 96, 97]. RAP/CVP is not an independent determinant ascertaining CO, and “depends on CO as much as it determines it” [98]. Accordingly, CVP can be low in a person with low blood volume and normal cardiac function, but it can also be low in cases of normal volume and good cardiac function [31]. CVP can be high in cases of normal filling with impaired cardiac function, but also in cases of normal function but with fluid overload [31].
Venous return depends on mean systemic filling pressure, right atrial pressure, and vascular resistance, particularly venous tone [98]. A rise in venus tone precipitates a progression in venous return. The pressure difference between the pressure in the periphery (systemic filling pressure which is largely represented by the pressure of the extrathoracic veins [99]) and the pressure within the right atrium (central venous pressure), is usually 7–10 mmHg whereupon RA-P is normally 0 mmHg, and determines the amount of venous return [100]. Spontaneous breathing, creating negative intra-thoracic pressures, increases this pressure gradient facilitating venous return [101]. On the other hand, increases in RAP and particulary elevated RAPs oppose venous return [98].
As the level of the right atrial pressure is decisively affected by extracardiac, intrathoracic conditions, any rise in pericardial pressure (displaying pericardial constraint, as found in case of pericardial effusion or tamponade, positive pressure ventilation and pleural effusions via heart–lung interaction) will enhance RAP [102, 103]. This rise in RAP is not accompanied by, or due to, an increase in filling volume, however it will further blunt venous return [98]. Increases in ventricular afterload and myocardial ischemia affecting diastolic myocardial properties (diminishing myocardial compliance) subsequently alter ventricular filling pressures and hence RAP (LAP respectively) [95, 104]. Accordingly, no relationship exists between RAP and SV in case extracardiac, intrathoracic pressures are elevated [103, 105].
As such, the level of the right atrial pressure is determined by the pressure with which the blood distends the atrium and by the pressure effects of the pericardial, thoracic, and pulmonary adjacent structures [103, 105].
Anyway, a high CVP may be related to (a) elevated extracardiac/intrathoracic features, or may (b) indicate RV-dysfunction and/or RV outflow obstruction (namely pulmonary hypertension PH) with blood welling up in the RA, or reflects (c) both a and b. In any case, differential diagnostic considerations are implicitly required as different causes will inevitably entail different therapeutic measures [98].
While in the arterial system the pressure depends on, and is determined by, the flow and the arterial resistance (MAP = SV × SVR) [1], the venous blood flow is determined by considering volume and venous capacitance [106]:


It is the venous capacitance which dominates the venous behaviour and the central venous pressure is determined, essentially, by the venous capacity [107, 108]. It is not the venous return (as a flow), but the volume that predominantly controls basic RAP/CVP [106].
During exercise, sympathetic activity, stimulated by the reduced activity from arterial and atrial receptors, will increase venous tone and decrease venous capacitance [109]. This will increase the venous return to the heart [110] and, in case of a recruitable preload reserve (this depends on CF [10–12, 75, 91, 94]), SV will increase [36, 37]. The immediate effect of a decrease in venous capacitance is an increase in all pressures [106], including transmural RVEDP and thus RV filling, enabling the RV to increase its systolic performance [36, 37].
Fluid infusion leads to an increase in venous capacitance, lowering the central venous pressure [108, 110, 111]. A high CVP always has consequences and will limit the venous return [49].
In patients with septic shock, Stephan [112] found that, despite vasodilatation of both the arterial and venous systems [113, 114], volume loading increased the venous tone and thus the CVP significantly and to high values (>10 mmHg). This is due to a marked reduction in the compliance of the venous system secondary to stiffening of the vein walls by several sepsis-induced mechanisms [112]. Furthermore, drastic increases in CVP indicte that the ability to accommodate in case venous return has reached its limit and that blood is welling up [98].
CVP is normally 0 mmHg at rest and might increase to 2–4 mmHg during exercise [115]. The CVP is only elevated in disease states [116, 117], a CVP > 10 mmHg often reflects an elevated RV-afterload [116].
In critically ill humans [4, 69, 93, 118, 119] as well as in healthy persons [76] we know that no correlation at all exists between CVP and preload or change in CVP and change in preload. The lack of a relationship is due to the fact that, in humans, the compliance of the atria and, in particular, of the ventricles is highly variable [76]. Furthermore, preload is not the same as fluid responsiveness [120, 121], and CVP and its change poorly (do not [122]) predict fluid responsiveness [10, 75, 123, 124].
Thus, in daily practice the absolute value of the CVP and even dynamic changes in its value are very difficult to interpret and cannot be used as a valid indicator of fluid management at all [117].
In general, a CVP ≥ 10–12 mmHg has to be considered high, and most patients within this range will not respond to volume administration [44]. Bafaqueeh [125] found that 40% of patients with a CVP < 6 mmHg did not respond to further fluid administration.
Pericardial constraint accounts for 96% of the RAP, if CVP > 10 mmHg [71]: A CVP ≥ 5 mmHg [126], and particularly when exceeding 9–10 mmHg, will exert substantial constraint on (left) ventricular filling [63, 127].
1.4 Hemodynamic Monitoring
1.4.1 Assessment and Monitoring of Fluid Status
Haemodynamic monitoring is a cornerstone in the management of critically ill patients [117]. It helps identify pathological states [13, 128] and complications of circulatory failure [13, 117] and aids restoration of normal haemodynamic parameters to prevent tissue and organ injury, to restore organ failure/dysfunction and hence to reduce mortality [117].
When faced with a compromised circulation, volume expansion is very frequently the first therapeutic measure used to improve haemodynamic status [129]. Unfortunately, only 40–70% of all patients with acute circulatory failure respond to fluid administration (SV/CO ↑) [75], which means that 30–60% of patients are not fluid responsive and volume administration may be harmful [117, 130–132]. Both, acute and chronic right heart failure [47] as well as acute left heart failure [23, 24] may deteriorate with volume loading.
Therefore a rational approach to fluid administration is needed, where the therapeutic decision is based on correctly assessed effective intravascular volume (preload) and the probable response to increased volume [117, 133]. However, the clinical tools available to evaluate the patient’s fluid status and specifically the intravascular/intraventricular filling (preload), such as jugular venous distension, crackles on auscultation, peripheral oedema, etc., are of minimal value and very poor indicators of the volume status, particularly in the critically ill patient with (cardiogenic) shock: They cannot be validated as a useful tool or basis for treatment decisions [134–138]. The only relevant clinical sign which, although still non-specific, may indicate a possible volume deficit is the heart rate. Volume deficits are usually compensated by an increase in heart rate (>90 bpm) to maintain CO in case of low SV [92, 139, 140].
In acute heart failure patients a two-minute bedside assessment [88, 141, 142] is extremely helpful to allocate the patient to one specific haemodynamic profile (wet or dry and cold or warm) with corresponding treatment regimes [88, 142–144] (see detailed information in Chap. 2). This evaluation, however, does not provide any usable information about the patient’s actual intravascular fluid status (to classify the patient as normo-, hypo-, or hypervolaemic) or whether a cold, and thus hypoperfused, patient will respond and benefit from fluids or not [28].
Hence, in addition to this useful bedside assessment, a proper assessment of the patient’s intra-vascular volume status must be carried out to clarify whether a benefit (positive fluid responsiveness) can be expected from volume expansion before fluids are given. Blind administration of intravenous fluid may be harmful through an increase in LVEDP [130], as the elevation of the LVEDP predominantly causes the patient’s symptoms to worsen [15] and, with increasing LVEDP, the patient’s prognosis [15, 16, 88].
In case of central hypovolaemia, volume administration will induce a significant increase in SV (flow) as long as a preload reserve can be recruited [130, 131, 145]. Thus, it is important to predict in a haemodynamically unstable patient whether this patient will increase his/her systemic blood flow (SV) in response to volume expansion or not [131].
Kumar [9] showed that, in healthy individuals, volume loading increases the systolic BP/LVESV ratio and the LV-SW by:
an increase in LV-SV due to a reduction in LVESV while the LVEDV remains unchanged and
an increase in contractility.
The contribution of the Frank–Starling mechanism is only mild to moderate, the contractility is the main component [9]. Kumar examined healthy volunteers and confirmed the findings of animal studies conducted in the 1960–70s [146–148]. Flow represented by SV is the original, central, and decisive parameter to be assessed when defining fluid responsiveness [75, 76, 121, 131, 145, 149].


In heart failure, although the LVEDV may be in the normal range, fluid administration can fail to increase the SV due to a significant reduction in contractility [10, 75, 91, 92]. Furthermore, we know that the Frank–Starling mechanism is impaired in heart failure [50, 90] and hence volume expansion may well be harmful and worsen the haemodynamic situation [117, 130–132].
Braunwald [91], and recently Michard [10, 75] have established proof that the increase in SV due to increased LVEDV depends on the contractility and pre-infusion preload (initial end-diastolic fibre length in respect to the Frank–Starling mechanism), particularly in the case of compromised cardiac function [10, 75, 91] (see Fig. 1.4).


Fig. 1.4
Δp: Change in pressure; ΔSV: change in SV; upper curve: normal heart function, lower curve; impaired heart function (modified from Michard, F [10], with permission)
In those patients with intermediate pre-infusion preload (normovolemia), the effect of volume loading depends exclusively on the contractility and, in the case of a compromised heart function (lower curve) in ‘intermediate preloaded’ patients, the effect of volume loading in order to increase SV, and thus CO and/or BP, is minimal and clinically not relevant [10].
Nevertheless, even in cases of cardiogenic or other types of shock, fluid administration may initially be helpful. Up to 70% of all patients in shock show a positive response (increase of blood pressure, increasing the perfusion of vital organs) when fluids are administered [150]. In non-responders we most often find that RV-dysfunction/failure with sepsis is the main underlying reason [150].
The physiological and pathophysiological facts described above demonstrate and emphasize that preload and fluid responsiveness are not the same, and this has been stressed in many published studies [117, 120, 121, 130, 151]. Therefore, as prerequisites to a positive response to fluid administration, there must exist both a recruitable contractile reserve (myocardial reserve) and an absolute or relatively hypovolaemic central vascular and cardiac system to provide a filling reserve.
1.4.2 Prediction of Fluid Responsiveness
1.4.2.1 Pressure Measurements
Cardiac filling pressures such as CVP and LVEDP/PCWP have failed to predict either preload or fluid responsiveness. The relationship (if there is any) between the intravascular/intraventricular volume and the CVP/PCWP is, as already mentioned, very poor in both ill patients [4, 69, 118, 119, 159] and healthy volunteers [76]. Even in sedated and mechanically ventilated patients, CVP and PCWP have been shown to be unreliable parameters to reflect the preload or to predict fluid responsiveness [10, 75, 76, 124, 160]. Osman [122] states that, “fluid responsiveness is documented to be unrelated to CVP/RAP and PCWP/LVEDP, respectively”.
1.4.2.2 Volumetric Measurements
Volumetric measurements (RVEDV, ITBV or GEDV) and ventricular areas (LVEDA or LVEDD) have been shown to be useful in assessing the preload and seem to be better than cardiac filling pressures in guiding volume therapy [75, 76, 161, 162] but, unfortunately, they are still not great at predicting fluid responsiveness [123, 163, 164].
In particular, it was hoped that GEDV(I), reflecting central blood volume [165, 166], and the direct measurement of the RVEDV would overcome the mentioned difficulties. However, the indirectly measured volumetric parameter GEDV failed to provide additional prediction in terms of the patient’s response to volume expansion [9, 161, 162, 167]. The direct measurement of the absolute value of the RVEDV allows a definitive assessment of volume status, however unfortunately whilst SV increased with volume loading there was no change in the measured RVEDV [9].
Furthermore, Reuter found only a poor correlation between SV and LVEDA (from echocardiography) [156], and Slama showed that changes in LVEDD are also dependent on LV stiffness [168]. Several other authors followed by confirming the poor correlation between LVEDD and SV/CO [123, 153, 159].
Thus, filling pressures such as CVP/RAP, PCWP, or areas/geometric dimensions of the LV, such as LVEDA or LVEDD, are unable to predict fluid responsiveness [75, 117], nor can direct [9] or indirect measurements of end-diastolic volumes (overview by [75]) predict the patient’s response to volume expansion [161, 162, 167].


Osman concludes that, in the assessment of preload responsiveness, parameters other than pressures and ventricular volumes need to be measured [122].
1.4.2.3 Dynamic Parameters
In contrast to the static parameters discussed above for assessing the filling pressures, filling volumes, and left ventricular areas, we have the dynamic parameters, which comprise stroke volume variation (SV-V), pulse pressure variation (PP-V), systolic blood pressure variation (SP-V) and aortic blood flow changes, which provide substantial information and are valuable tools in predicting fluid responsiveness [123, 140, 152, 153, 162, 169].
The dynamic parameters reflect changes in LV-SV due to heart-lung interactions induced by mechanical ventilation [123, 139, 167, 170, 171] and several studies have documented that variations in LV-SV associated with mechanical ventilation are highly predictive of preload responsiveness [152, 153, 156, 158, 168].
The alterations in cardiac preload, and hence variations in LV-SV associated with respiration, are referred as to SV-V and are defined by the maximum to minimum SV values during a period of three breaths, or over a time interval of 20–30 s [153, 158, 168]. SV-V is validated in several studies for deeply sedated, mechanically ventilated patients with a tidal volume of 6 mL/kg without any spontaneous breathing effort. A SV-V ≥ 10% predicts an increase in CO of ≥15% for a 500 mL fluid bolus [157, 158, 168].
Positive pressure ventilation with its cyclic increases in intrathoracic pressure and lung volume [172, 173] induces intermittent variations in cardiac preload (heart–lung interaction) [156, 174–176]. This is predominantly due to a reduction in venous return secondary to the increase in RA pressure during mechanical inspiration [174, 177–179]; hence, the RV filling is reduced (↓ RVEDV) [174, 180–182]. In accordance with the Frank–Starling mechanism this produces a reduction in RV-SV [36, 37, 183]. An additional effect that is at least partly responsible for the reduction in RV-SV is exerted by the increase in RV-outflow impedance [184, 185] and thus a rise in RV-afterload with consecutive impaired RV ejection secondary to positive pressure ventilation [176, 186–188].
However, this inspiratory reduction in RV-SV affects the LV-filling after a few heart beats, producing a ↓ LVEDV [175, 189, 190]. Consequently, the LV-SV is reduced [175, 176, 189, 190] and this takes effect during expiration. Thus, ventilation-dependent variations in RV-filling will induce cyclic variations in LV-filling with a concomitant reduction in LV-SV, and thus arterial blood pressure, if both RV and LV are fluid responsive [117, 176, 183, 189].
Conversely, during inspiration the opposite occurs; increased LV-filling will result in a higher LVEDV and hence higher LV-SV and arterial pressure [117, 176, 189].
The influence of positive pressure ventilation on the cyclic haemodynamic changes is greater when central blood volume is low rather than when it is normal or high [75, 135, 153].
The dynamic parameters will lose their validity if tidal volumes vary from breath to breath, as with (assisted) spontaneous breathing [131, 155, 191] or in case of marked arrhythmias inducing variations in LV-SV [135]. Exaggerated values of SV-V were found with large tidal volumes, reduced chest wall compliance and air trapping [156]. Furthermore, a moderately elevated intra-abdominal pressure (up to 20 cm H2O) affects cyclic circulatory changes by inducing a progressive increase in intrathoracic pressure enhancing the pleural pressure swings and thus may feign fluid responsiveness [192]; if the intra-abdominal pressure is higher than 20 cm H2O, less influence is seen [192].
Nevertheless, the dynamic parameters have shown themselves to be far better than the static parameters in predicting fluid responsiveness and are currently the approach of choice in sedated and ventilated patients [117, 135, 152, 153, 155, 156].
The dynamic swing in LV-SV is the current gold standard [145, 152] in predicting response to fluid administration—but SV-V, although affected by preload, predominantly also seems to reflect the myocardial response to volume loading [156]. This is consistent with our knowledge that SV predominantly depends on LV-function (mainly the contractility [10, 75, 91, 92] and, in heart failure, on afterload as well [11, 12, 46, 94]) rather than on pre-infusion preload [10, 75, 91]. Kumar [9] showed that, in healthy volunteers, the increase in SV due to volume loading is predominantly a result of an increase in contractility rather than an increase in filling volume, and thus a larger fibre stretch as described by Frank and Starling.
Besides the assessment of SV-V during positive pressure mechanical ventilation [191], surrogates of SV such as aortic flow [153, 157], systolic BP (SP-V) [189, 193], and pulse pressure (PP-V) [145, 183] have turned out to be reliable and valuable indices by which to check central blood volume and the response to fluid administration.
Descending Aortic Blood Flow as a Direct Correlate of SV/CO
Descending aortic blood flow represents the majority of CO [194, 195] and is accepted as a clinically realistic estimate of SV and or CO [196–198]. Aortic Doppler flow velocity measurements can determine the SV, calculated with the help of the product of the velocity-time interval in the ascending (estimated by echocardiography [151]) or descending aorta (oesophageal Doppler measurement) [197, 199, 200] and a measured [151] or estimated aortic diameter using the nomogram by Boulnois [195]. These flow velocity measurements have been reported to predict fluid responsiveness accurately [153, 168, 197].
Systolic Pressure Variation (SP-V)
Systolic pressure variation (SP-V) is probably the easiest way to assess fluid responsiveness and is defined as an ‘increase or decrease in systolic arterial pressure with each mechanical breath relative to the systolic pressure during the short apnoea phase’ [193, 201]. Numerous studies have shown its value as a sensitive parameter in predicting preload responsiveness in patients who are mechanically ventilated without any spontaneous breathing [123, 153, 156, 167, 169, 202]. The sensitivity of this method is not as high as that of PP-V because it does not quantify the varying diastolic arterial pressure components [183].
Pulse Pressure Variation (PP-V)
Pulse pressure variation (PP-V) may be the most robust and sensitive indirect indicator of volume status [75, 183]. The variation of the aortic pulse pressure (aortic pulse pressure ~ LV-SV [203, 204]) is established as an evidence-based index with which to assess and predict the response to fluid administration in mechanically ventilated patients [75]. A cyclic variation of the aortic pulse pressure due to varying LV-SV during a respiratory cycle of more than 13% (r2 = 0.85, p < 0.001) [75] implies a very high likelihood (85%) that the patient will benefit from fluid administration with a significant increase in SV and thus in blood pressure (positive predictive value of 94%, negative predictive value of 96%) [75, 190, 205].
Calculation of PP-V during one respiratory cycle:
![$$ \begin{array}{l}\mathrm{Ppmax}:\ \mathrm{maximal}\ \mathrm{systolic}\ \mathrm{pressure}-\mathrm{maximal}\ \mathrm{diastolic}\ \mathrm{pressure},\\ {}\mathrm{Ppmin}:\ \mathrm{minimal}\ \mathrm{systolic}\ \mathrm{pressure}-\mathrm{minimal}\ \mathrm{diastolic}\ \mathrm{pressure}.\\ {}\mathbf{PPV}\left(\%\right)=\left[\right(\mathbf{Ppmax}-\mathbf{Ppmin}\Big)/\Big(\mathbf{Ppmax}+\mathbf{Ppmin}\Big)/\mathbf{2}\Big]\times \mathbf{100}.\end{array} $$](https://i0.wp.com/thoracickey.com/wp-content/uploads/2017/09/A183660_2_En_1_Chapter_Equi.gif?w=960)
![$$ \begin{array}{l}\mathrm{Ppmax}:\ \mathrm{maximal}\ \mathrm{systolic}\ \mathrm{pressure}-\mathrm{maximal}\ \mathrm{diastolic}\ \mathrm{pressure},\\ {}\mathrm{Ppmin}:\ \mathrm{minimal}\ \mathrm{systolic}\ \mathrm{pressure}-\mathrm{minimal}\ \mathrm{diastolic}\ \mathrm{pressure}.\\ {}\mathbf{PPV}\left(\%\right)=\left[\right(\mathbf{Ppmax}-\mathbf{Ppmin}\Big)/\Big(\mathbf{Ppmax}+\mathbf{Ppmin}\Big)/\mathbf{2}\Big]\times \mathbf{100}.\end{array} $$](https://i0.wp.com/thoracickey.com/wp-content/uploads/2017/09/A183660_2_En_1_Chapter_Equi.gif?w=960)
Passive Leg Raising (PLR), An Autotransfusion of Fluids
Several studies recently published have given encouraging evidence that prediction of fluid response is feasible in spontaneously breathing as well as ventilated patients [130, 151].
Raising the legs to approximately 30 or 45° is called passive leg raising (PLR) and will increase the aortic flow in case of a recruitable preload reserve 15–60 s after the legs have been raised [131, 145, 149, 151] and this will persist for 30–90 s [206] (Pinsky [117] up to 3 min).
Clinical studies have proven that the volume of blood transferred to the heart by PLR is sufficient to increase the left ventricular filling volume [131, 145, 207, 208]. While the predictive value of the transient changes in SV is only fair if SV or its surrogates, SP-V and PP-V, are estimated from a peripheral pulse pressure curve [129, 131]—due to the influence of the arterial compliance and the vasomotor tone [145, 204]—high sensitivities were achieved when measuring variations in SV centrally, i.e. by oesophageal Doppler [131], echocardiography [151] or by femoral artery access, which is considered to be central [209, 210]: Monnet [131] found a sensitivity of 97% and a specificity of 94% to achieve an increase of ≥15% in aortic blood flow in response to volume administration if, during PLR, the aortic blood flow increased by ≥10%. Lamia [151] showed a similar specificity (100%) but with a slightly worse (but still good) sensitivity of 77%.
Thus, an increase in aortic blood flow (SV/CO) by ≥10% [131, 145] or 12.5% [151] during PLR is reliably predictive of central hypovolaemia and a positive response to volume expansion [130, 131, 145, 151] in either mechanically ventilated patients or those breathing spontaneously. In the absence of central hypovolaemia and/or in the presence of an unresponsive RV and/or LV (compromised function, mainly impaired contractility) SV/CO will not increase by the PLR manoeuvre [131, 145].
As no external fluids are administered, the hazards of unnecessary volume loading can be avoided [44, 87, 162, 211–213] and hence the measurement of central blood flow (aortic blood flow normally represented by SV or CO) in response to PLR is more robust and probably superior to PP-V when evaluating the patients’ fluid response, even in spontaneously breathing patients [121, 130, 131, 151]. Furthermore, this approach is more independent of varying tidal volumes and arrhythmias than a peripheral one [130, 131, 151]. The central measurement of blood flow avoids the relevant influences of arterial compliance and vasomotor tone [204] and the complex changes in pulse wave propagation and reflection along the arterial vessel system [214], both of which may change during PLR with a concomitant change in SV.
1.4.2.4 Fluid Challenge
A fluid challenge is still advocated as a tool to evaluate the need for further fluid administration if strictly monitored and the response observed closely [133, 215], but the dynamic parameters described above are clearly superior and blind volume administration should be avoided if at all possible [130].
A fluid challenge does not mean fluid resuscitation; it merely identifies those patients who are likely to show a beneficial response to (further) fluid administration [216]. To minimise the amount of fluid needed to assess responsiveness, the fluid should be given quickly [44] and some authors require an increase in CVP of at least 2 mmHg [217, 218] to confirm that a sufficient amount of fluid has been given. Rapid bolus administration of 250 mL in 5–7 min or 500 mL in 10 min [44] of fluid or PLR is expected to show an appropriate haemodynamic response if beneficial for the patient [116, 217]. If a recruitable preload reserve is available, the SV must increase [217].
Although no definition as to what comprises an adequate fluid challenge is generally agreed upon, most studies do agree that a positive response is indicated by improving circulatory status as suggested by ↑ BP, heart rate unchanged or ↓, with accompanying SV ↑, and an improved effective blood flow documented by ScvO2/SvO2 ↑, and lactate ↓ [116].
It is always worth remembering that a fluid challenge should only be performed if an indication is obvious, i.e. within the context of hypoperfusion [219] and that there is only a very poor correlation between change in BP and CO [44]. If no positive effect is achieved, fluid administration is useless, potentially harmful, and must be stopped immediately [44, 87, 162, 211–213].
Despite uncertainty, even in life-threatening situations such as cardiogenic shock, the administration of moderate amounts of fluid (about 3 mL/kg, hence ~ 250–300 mL) as a fluid challenge under close monitoring is appropriate and may stabilize the acute situation temporarily [220].
Appropriate and immediate fluid resuscitation in critically ill patients, if adequate, will improve outcome [221]. McConachie [222] states that a fluid challenge is appropriate in virtually all critically ill patients in shock situations with blood pressure ‘too’ low and/or hypoperfusion due to low cardiac output, unless obviously suffering from gross congestive cardiac failure.
On the other hand, it must be emphasised that, although a patient responds to volume administration, this does not automatically mean that the patient requires volume, as healthy subjects will respond as well [44, 216].
Vincent and Weil have recently proposed the following algorithm as being the proper approach to performing a fluid challenge [133]. In hypotensive patients with circulatory compromise administer 250–500 mL colloidal fluid (~3–5 mL/kg) over 15–20 min in order to stabilize the patient haemodynamically (at least temporarily), to improve organ and tissue perfusion, and to ‘test the system’ as to whether or not they are likely to respond positively to further fluid administration.
Criteria suggestive of effective volume loading [10, 31, 133, 183, 219]:
increase in SV by ≥10% and/or increase in systolic blood pressure by ≥10%,
heart rate unchanged or reduced,
CVP increase ≤ 2–5 mmHg (if >5, no further administration, be cautious already if increase >2),
no clinical signs of fluid overload,
additional parameters, if monitored:
PCWP increase ≤ 3–7 mmHg; stop fluids if increase >7 mmHg,
EVLWI prior and post fluids ≤ 7–10 mL/kg,
↓ lactate, positive result by OPS (see below),
increase in urinary output.
Stop fluid challenge during or after infusion if [10, 31, 133, 183, 219]:
Hypoperfusion does not improve (clinically, no ↑ UO, no ↓ lactate / no ↑ SaO2, no change in capnography/OPS evidence of improved tissue perfusion);
CVP increase > 5 mmHg due to volume administration, be cautious if increase > 2: ↑ risk for DVI;
High risk of DVI if CVP > 9–10 mmHg [116, 117, 127, 223] and particularly if SV/BP falls during volume administration.
An International Consensus Conference [218] from 2006 suggested ‘a rise in CVP of at least 2 mmHg either by 250 mL fluid administration within 10–15 min, or leg raising’ as a sign of sufficient fluid administration—defining a positive response if cardiac function and tissue perfusion improve. However, bear in mind that this recommendation is non-specific and expert opinion only.
As we know, CVP does not reflect preload or changes in preload, either in healthy or critically ill patients [69, 76, 119, 144, 159]. Thus, CVP cannot be used as a predictor of RV-filling and cannot be used to assess the effect of volume loading. A change in the magnitude of the CVP of at least 2 mmHg is the minimum necessary for detection with confidence on most currently used monitors [44] and therefore seems to be an arbitrary figure. Remember, in patients with good cardiac function, the CVP may even fall despite the fluid challenge being successful [76] and, if using the PLR method, central monitoring is essential and peripheral monitoring is not adequate [129].
1.4.2.5 PiCCO-Monitoring (Pulse-Induced Continuous Cardiac Output)
PiCCO is a method of haemodynamic monitoring which combines transpulmonary thermodilution and continuous arterial pulse contour analysis (see overview by Pfeiffer [227]).
This method allows the measurement of volumes [34, 160, 228] such as intra-thoracic blood volume (ITBV) representing the intra-vascular volume status, the global end-diastolic volume GEDV (of all four chambers) and, of most importance, the extra-vascular lung water (EVLW) [224, 229].
These volumetric measurements are performed semi-invasively and are superior to the common pressure measurements, CVP and PCWP, when assessing the patient’s intravascular volume status and the cardiac preload [84, 85, 119, 230]. Unfortunately, these parameters (ITBV and GEDV) do not allow any prediction of the response of the circulatory system to fluid administration [75, 161, 162] (see above). However, the PiCCO-method fulfils all the requirements to evaluate response from PLR [131, 151, 231].
EVLW is an extremely informative parameter, proven as being an accurate measurement of the real amount of fluid in the lung tissue [225, 229, 232], the EVLW value provides substantial information about patient prognosis [211, 224, 232]. Currently, it is the only method able to diagnose ‘developing’ pulmonary oedema earlier than all other available methods, including clinical examination, chest X-ray and pressure measurement via PA-catheter (PCWP) [119, 233–236]. Furthermore, it is able to guide investigation of the pathologically high lung water: cardiac or extra-cardiac causes [213, 229, 237].
Two-thirds of all HF patients with a mean PCWP of <18 mmHg (18 mmHg is the generally accepted upper limit in case of a failing heart, probably providing the maximum preload recruitable SV) show a significantly increased EVLW/EVLWI [238], although it is not detect- able by auscultation or on X-ray [239, 240]. On the other hand, the PCWP is measured to be normal (≤12 mmHg) in some cases of cardiogenic shock, particularly in previously healthy patients with acute myocardial infarction, but the EVLW is already elevated and thus pulmonary oedema is present [28, 241, 242]. An increased EVLW/EVLWI signals increased mortality [211, 232, 243] and in the case of an elevated EVLW, any fluid reduction will lead to an increase in CO [234] (Fig. 1.5).


Fig. 1.5
This diagram shows the mortality rate depending on the amount of extravascular lung water. The graph is of special value because it is validated by post mortem analysis of lung water, confirming the accuracy of the clinical measurement (adapted from Sturm et al. [232]), with permission
EVLW is valuable in indicating fluid overload [225, 235, 236] and its value (normal range EVLWI 3–7 mL/kg) should influence your therapeutic decision. If the EVLWI exceeds 10 mL/kg, the mortality increases exponentially and further fluid administration is not advisable [212, 226, 232, 243].
The permeability index PVPI (PVPI = EVLW/pulmonary blood volume (PBV) with PBV = ITBV − GEDV) reflects, if elevated (>3), an increased capillary permeability (capillary leakage resulting in non-cardiogenic oedema) [213, 229, 237], while an index <3 in combination of elevated EVLW/I is suggestive for a cardiogenic oedema.
1.4.2.6 Echocardiography
Echocardiography is essential to help diagnose the underlying pathology in circulatory failure and/or cardiac dysfunction [244, 245]. Heidenreich [246] successfully improved diagnostic accuracy by identifying a further 28% of the underlying aetiologies in unexplained hypotension when examining patients by transoesophageal echocardiography (TOE) in addition to the other obtained hemodynamic parameters. Thus, he showed that TOE adds significant information to invasively acquired haemodynamic data. Echocardiography has the ability to rapidly diagnose and aid decisive therapeutic decisions in cases of cardiac tamponade [247] and aortic dissection [248], confirming the clinically suggested diagnosis of endocarditis [249], to reveal evidence of haemodynamically significant pulmonary embolism [250], and is, of course, extremely helpful in assessing the heart’s performance [251].
The assessment and evaluation of SV/CO, probably the main determinant of sufficient organ perfusion, is relatively easy to obtain by flow measurement in the descending aorta [200]. Laupland [199] gave proof that this is easy, quick to learn, and simply done in daily practice. However, this method does have some limitations. It is assumed that about 70% of the total CO will reach the descending aorta [195] and, furthermore, instead of measuring the diameter of the LVOT needed for the calculation of CO, a nomogram by Boulnois [195] is used. Thus, this method provides a rough estimate of the CO and the correlations with invasive measurements are weak when compared with PA-catheterisation or PiCCO [243, 252, 253]. If estimating the CO with PA catheterisation, as recommended by the ESC and AHA, advanced skills and training are necessary [254].
There have been 11 large studies evaluating the use of echocardiography as a continuous monitoring method in critically ill patients, most of them using the transoesophageal technique. No final conclusion can be made as to whether or not echocardiography should be recommended as equal to the established methods in continuous haemodynamic monitoring.
Echocardiography is time consuming, requires advanced physician training in acquisition and interpretation, and it is not realistic to establish this technology on a 24 h basis worldwide [176, 255, 256]. The usefulness of echocardiography lies in its diagnostic capacity and there is a consensus that an echocardiogram is absolutely essential in the initial assessment of all patients suffering from (cardiogenic) shock and should be performed as early as possible [244, 245, 257, 258]. Echocardiography (especially TOE) frequently depicts abnormalities overlooked by catheter-based invasive assessment tools such as LVOT obstruction, diastolic ventricular interaction, RV-dysfunction/failure, LV diastolic dysfunction, valve disease, cardiac compression, etc. [246, 259]. Furthermore, it has a great impact on therapeutic considerations, with 60% [260] of planned treatments altered following echocardiography [261–264].
Echocardiography can be a life saving tool; in cardiac failure patients, echocardiography is far easier and faster than PA-catheterisation and provides key haemodynamic information [265].
1.4.3 Arterial Blood Pressure
1.4.3.1 BP and Autoregulation
Adequate organ perfusion is essential to avoid the development of shock [266]. Although the mean arterial pressure (MAP) is the best estimate of organ perfusion pressure [116], there is no known threshold pressure defining adequate perfusion pressure amongst different organs, between patients, or in a patient over time [267]. The autoregulation of most organs maintains a constant organ-specific blood flow over a broad range of varying BPs and changes in metabolic rates, but hypotension is always pathological [116, 117].
Most authors define hypotension as systolic BP < 90 mmHg [268, 269], MAP ≤ 65 [267, 270] to 70 mmHg [271, 272], although in known hypertensive patients this may be altered to a MAP ≤ 85 mmHg and, in known hypotensive patients, ≤50–60 mmHg. In patients with IHD a MAP of ≤75–80 mmHg [267, 273–275] is commonly used.
Hypotension impairs autoregulated blood flow distribution [276, 277], and the MAP needed to maintain autoregulation varies from organ to organ and depends on clinical conditions (i.e. known arteriosclerotic disease or not).
Kidneys
A constant renal blood flow is maintained by autoregulation, which acts in a range of MAPs between 80–180 mmHg [278–280]. Iglesias [281] demands a MAP > 70 mmHg in order to prevent acute renal failure, or if acute kidney injury has already developed, in order to re-establish adequate renal perfusion. Esson [282] stresses that adequate renal perfusion pressure is a cornerstone of care in acute renal failure.
Brain
Autoregulation works within MAPs of 60–160 mmHg [283], the recommendations for an adequate cerebral perfusion pressure in critical illness vary from at least 60 mm Hg [284, 285] to ≥70 mmHg [283, 286–288].


(In case of brain injury even higher pressures may be desirable).
Heart
A coronary perfusion pressure (CPP) is determined by:


[289]
Coronary autoregulation functions from (50 [273]) 60 mmHg up to 140 mmHg [273, 274]. This means that in the case of an elevated LVEDP (>15 mmHg), a minimal diastolic pressure of > 65 mmHg is essential. In coronary artery disease, even higher pressures are required in order to prevent further deterioration due to progressive ischaemia [267, 273–275].
Septic Shock
In septic shock, a MAP between ≥ 65 mmHg [260, 270, 275, 290] and 75 mmHg (in patients with known occlusive arterial disease, peripheral arteriosclerosis or long standing hypertension) [275] is recommended. A study by LeDoux showed that a MAP between 65 mmHg and 85 mmHg was not associated with significant differences in organ perfusion [267].
This was confirmed by Bourgoin [291] who showed that an increase in MAP from 65 mmHg to 85 mmHg with an infusion of noradrenaline did not improve renal function. The key point is that, as long as autoregulation is not substantially disturbed, a MAP of ≥65 mmHg is sufficient. But in case of a breakdown of autoregulation, however, higher MAPs are necessary to re-install it [270].
1.4.3.2 Assessment of Tissue Perfusion
Organ perfusion essentially depends on blood flow and thus cardiac function [200]. Circulatory shock is known to cause tissue hypoperfusion [117] and inadequate tissue perfusion is associated with elevated morbidity and mortality [221, 294–298].
Compared to the difficult task of evaluating the vascular fluid status and the patient’s likely response to volume expansion, tissue hypoperfusion can be assessed fairly well by clinical examination [257, 269, 299]. Clinical signs suggestive of tissue hypoperfusion are [129, 130, 151]:
tachycardia,
hypotension (sBP < 90 mmHg, MAP < 70 (60) mm Hg, or BP-drop > 40 mmHg),
oligo-/anuria,
clinical or biological signs of extracellular fluid depletion (ketoacidosis, vomiting, diarrhoea),
delayed capillary refill,
mottled skin,
altered level of consciousness.
Menon [257] strongly recommends a diagnosis of cardiogenic shock (CS) in all patients exhibiting signs of inadequate tissue perfusion in the setting of severe cardiac dysfunction irrespective of the BP.
SvO2 (mixed venous oxygen saturation) reflects the balance between oxygen delivery and oxygen consumption [291, 300]. Pinsky [117] and Reinhart [301] state that a decrease in SvO2 to <70% represents increased oxygen extraction by the tissues [117, 301] suggestive of hypoperfusion [302]. A persistent SvO2 < 30% is associated with severe tissue ischaemia [303].
Plasma lactate levels, although non-specific, are still a reasonable surrogate for inadequate tissue perfusion [304, 305]. A reduction of an initially elevated value signals improvement of perfusion [306].
Thus, ↑ plasma lactate levels and ↓ SvO2 [307, 308] coupled with a suggestive clinical examination may help support the earlier diagnosis of tissue hypoxia.
Ander [309] found that monitoring of ScvO2 and lactate in patients with severe heart failure (patients with known cardiomyopathy being admitted with acute decompensation) is superior to assessment and monitoring clinical vital signs for the recognition of occult cardiogenic shock. If both parameters are abnormal (lactate > 2 mmol/L, ScvO2 < 60%), occult/pre-cardiogenic shock requiring a special therapeutic approach could be clearly identified, whilst this was not possible from the vital signs [309].
Newer developments such as sublingual capnography [310], orthogonal polarization spectral spectroscopy (OPS) [311, 312] and near-infrared spectroscopy (NIRS) attempt to measure local tissue blood flow and oxygen utilization [287, 313] and evaluate any improvement due to therapeutic intervention.
Due to the fact that the use of ‘the conventional global haemodynamic and oxygenation approach’ may fail to provide adequate information on tissue perfusion, non-invasive monitoring of peripheral perfusion could become complementary in acting to warn of imminent global tissue hypoxia [314].
It must be remembered that the rationale for haemodynamic monitoring is to restore normal haemodynamic parameters in order to prevent organ injury and restore organ dysfunction [117], however this may not be valid in all cases. Haemodynamic monitoring usually assesses the global circulatory status, not organ function or microcirculation [288, 315–319], and does not address the mechanisms by which disease occurs [320, 321]. Therefore, we have to be careful in drawing therapeutic conclusions from the results of monitoring the macrocirculation, improvement of macrocirculation may compromise the microcirculation even further [322].
1.5 Afterload
1.5.1 Definition
1.5.2 Vascular Properties, Effective Arterial Elastance, Wall Stress and the Law of LaPlace
Braunwald [30] states: “the load opposing LV ejection, in its simplest sense, is reflected by the systolic blood pressure”. However, the physiology is much more complex and systolic blood pressure has turned out to be a very poor reflection of afterload. Indeed, the arterial system imposes a hydraulic load on the heart, and a higher arterial load requires higher energy to eject a given amount of blood. This vascular, hydraulic load, opposing ventricular ejection is most completely described and reflected by aortic input impedance1 (respectively pulmonary artery input impedance) [326–329].
The main parameters characterizing arterial input impedance are peripheral vascular resistance, total arterial compliance, and aortic characteristic impedance [326, 329, 331]. While peripheral vascular resistance specifies steady state conditions, the pulsatile load (pulsatile load is complex and time varying [332]) components are represented by:
- 1.
The total arterial compliance, reflects, by quantifying the pressure-volume–relation, the overall structural behaviour of the arterial system as a whole [327, 333–335], but specifically represents properties related to pulse wave propagation and reflection [327, 336–338], which affects the loading conditions, as intensity and timing of the pressure wave reflections are influenced by inertial forces, and oppose LV ejection [339], and
- 2.
The characteristic impedance outlines physical properties, such as viscoelasticity and dimensions of the large central, proximal arterial vessels (aorta, respectively pulmonary artery), and thus the contribution of elastic vascular properties to total load [327, 328, 340, 342, 343]. “Pulsatile afterload” largely includes characteristic impedance and pulse wave reflections [326, 340, 344], directly opposing ventricular ejection.
In fact, special attention has to be paid to the impact of the pulsatile elements on the total vascular load, as the intensity and the timing of reflected pressure waves change according to the elastic vascular properties (largely proximal aorta), and thus may exert a substantial impact on the vascular load the ventricle is facing [338, 345, 346]. In case of arterial vascular stiffening, as occurring with (physiological) ageing [347–349] or in hypertensive individuals [317, 350, 351], the wave velocity increases, and reflected waves return and sum up with incident forward waves, augmenting net pressure [318] and reaching the ventricle (already) in late systole, after-loading the ventricle [319, 338]. Concomitantly, aortic input impedance considerably increases [317, 319, 352, 353]. Accordingly, reflected pressure waves are shown to exhibit a substantial impact on systolic load imposed on the heart [345], and arterial stiffening is recognized to afterload the ventricle [317, 319, 354], by elevating (late) systolic load, thereby increasing systolic ventricular elastance, compromising ventricular filling, and influencing diastolic properties with raising filling pressures [347, 355]. Indeed, it has to be emphasized, that diastolic cardiac function is affected if arterial compliance decreases as in arterial stiffening [356]. Therefore, central vascular stiffening and pulse wave reflections determine late systolic arterial loading [338, 346].
The impact of pulsatile load, particular wave reflections, is even more relevant in the pulmonary circulation [341, 357]: In contrast to systemic circulation, resistance and compliance of the pulmonary vasculature are inversely releated to each other, and are evenly distributed over the complete vessel tree [358, 359]. Accordingly, an elevated pulmonary capillary wedge pressure, by decreasing pulmonary vascular resistance, enhances net RV afterload, due to increasing pulsatile load relative to the resistive one [357].
However, it is hard to obtain aortic (pulmonary artery) input impedance, as a frequency domain analysis (by Fourier method) of simultaneously measured pressures and flows is required [328, 360, 361] in order to describe the relation between arterial pressure and flow within a vessel/vessel system [327]. This is a technical challenge [360, 361] and additionally, it would be quite complicated to apply the derived frequency domain factors to daily clinical concepts and routines [330]. Sunagawa [362] made vascular properties (evaluated in the frequency domain) comparable with ventricular properties (expressed in the time domain), by lumping principal elements of vascular load (peripheral vascular resistance and total arterial compliance, characteristic impedance, and systolic and diastolic time intervals) together in (effective) arterial elastance (Ea), and as such, constitutes a close approximation of arterial load [363]. Effective arterial elastance characterizes aortic input impedance, and thus arterial load that is imposed on the ventricle [362]. This “simple measure”, which lumps together static and dynamic components of impedance, has been shown to perform well in experimental studies [362, 364]. Although dominated by the non-pulsatile load component (SVR), Ea is also altered by artery stiffening due to increased pulsatile load [365]. Ea is the most complete, and also reasonably applicable, delineation of aortic input impedance [330].
Operationally, Ea is numerically defined as the ratio of end-systolic ventricular pressure to stroke volume, and is directly related to heart rate and peripheral vascular resistance, and is inversely related to total arterial compliance (which is determined, in large parts, by the central elastic arteries) [366]:


However, practically, Ea is derived from the pressure-volume relation, defined as the ratio of left ventricular end-systolic pressure (LVEDSP) to left ventricular stroke volume (LV-SV ) [362, 363]:


This equation can be further simplified: If LVESP equals systolic arterial pressure (sBP), corrected by 0.9 [363], Ea may be calculated as: Ea = LVESP/LV-SV = sBP × 0.9/LV-SV.
Regarding the right ventricular—pulmonary vessel system interaction, Ea-Pulm is reported to be a reliable measure of the load faced by the RV during systole, and accounts for pulmonary vascular resistance, compliance, and impedance, thus including pulsatile components of arterial load [341, 370, 371].
However, the tension the ventricular wall sarcomeres must overcome during systole in order to shorten is related to:
Accordingly, aside from the vascular properties opposing and affecting ejection, there are specific cardiac properties contributing to, and participating in, afterload characterization. As such, myocardial wall stress during contraction represents “true” afterload, because wall stress reflects both central aortic and peripheral, vascular loading conditions and intrinsic heart muscle properties, such as LV geometry, LV size and intra-cavitary pressure [324, 375–377].
The relation between afterload and systolic ventricular wall stress can be formally defined by the law of Laplace [329, 378–381]: ơ = p x r/2h
(ơ represents wall stress, p = ventricular pressure, r = LV or RV radius and h = wall thickness).
(The law of LaPlace applies to spherical figures, thus its transposition and application on the right ventricle with its varying regional internal radius may be problematic [380]).
Thus, directly applied:


[382]
Dilatation will induce an increase in LV(RV) diameter and generally in LV (RV) filling pressure, and as such leads to a rise in wall stress. An increase in wall thickness (in the case of hypertrophy) reduces the wall stress.


Determinants of the LV wall stress mediated by LaPlace’s law are continuously changing during systole, producing varying measurements of LV wall stress depending on the phase of the cardiac cycle. Peak wall stress occurs within the first third of ejection, and wall stress then declines to its end-systolic value, which is less than 50% of the peak value. At the same time, the total systolic wall stress (estimated by the stress time integral), predicts myocardial oxygen consumption [384].
All measures show a significant difference and the choice of index depends on the question being asked [384]:
total stress reflects myocardial oxygen consumption,
peak stress correlates closely with the progress of hypertrophy, and
end-systolic wall stress represents most accurately the afterload.
The very good correlation between end-systolic wall stress and myocardial fibre length at end-systole [69], as well as between end-systolic wall stress and end-systolic ventricular volume (ESV) [385–387], underlines the fact that the end-systolic wall stress is literally the (after)load that limits the ejection [388, 389].


Furthermore, several authors have confirmed the excellent correlation between end-systolic wall stress and LV afterload in daily practice [323, 376, 386, 387, 390].
During systole, the LV-chamber size will decrease while the ventricle contracts, and thus the wall tension will fall. When the afterload increases, a greater rise in pressure is necessary for any given reduction in chamber size, and therefore, wall tension during systole is higher. The pressure increase has to be even greater, of course, in a primarily dilated LV [19].
By the way, there are two echocardiographic methods described by Reichek [378] (M-mode assessment, meridional wall stress) and Greim [391] (2D-assessment, circumferential wall stress), which directly assess the end-systolic wall stress. Both are time consuming, require advanced skills, and Greim [391] expresses concerns about the ability of the M-mode method to recognize acute changes in afterload in patients during cardiothoracic surgery.
To summarize, two alternative biophysical concepts may describe and characterize afterload [329]. Ross and co-workers [392] gave evidence, that the level of wall stress, rather than input resistance or pulsatile impedance, determine ventricular performance, favouring wall stress as the most exact feature representing “true” afterload. Furthermore, arterial input impedance specifically refers to vascular properties, while more or less neglecting cardiac properties. As such, while wall stress integrates the forces that oppose ventricular ejection, Ea is a measure of the hydraulic load faced by the ventricle [380, 393]. Wall stress is considered to be the most accurate feature to describe ventricular afterload [329]. However, while wall stress estimation has not gained any clinically feasible relevance in daily practice, Ea may be obtained with reasonable effort.
1.5.3 Afterload Mismatch and Acute Heart Failure Syndromes
In order to perform well, heart performance and afterload have to match, leading to the concept of afterload mismatch [329]: Basically, “a mismatch can be induced acutely in a normal heart if end-diastolic volume is not allowed to compensate for the increase in afterload” [394]. Subsequently, SV, EF and ventricular circumference will fall [329]. Examples include: volume depletion in the presence of rapid and substantial rise in systemic pressure, and increases in afterload in a ventricle already having utilized the maximal preload reserve with average sarcomere length exceeding 2.2 μm, indicating maximal stretch (limited by pericardial constraint, and as such explaining why there is no descending limb of the Starling curve). Imposing an extra load on such a ventricle will cause a sharp drop in SV, unless the contractility can be increased intrinsically or by applying inotropic agents [395].
1.5.4 Concluding Remarks
Afterload can be defined as the forces that oppose ventricular muscle fibre shortening [329, 396]. Features opposing comprise:
Systolic wall stress integrates the forces opposing ventricular ejection, accordingly, wall stress may be considered the most accurately feature characterizing the load faced by the ventricle during systole [324, 332, 375–377, 397].
However, the clinical feasibility to apply the one, or the other, method assessing the highly complex relationship between ventricular contraction, arterial system and blood flow at bedside is nearly impossible [329]. Furthermore, that one single parameter can fully encompass all aspects cannot be expected [329]. To simultaneously measure pressures, wall thickness and radius in a constantly changing system, in order to determine wall stress, is currently not feasible. Therefore, simplified derivations are necessary and as such, effective arterial elastance has gained high acceptance and is widely used, at least in medical research, to evaluate the discussed relationships, and thus get insights into pathophysiological and pathogenetic processes, interrelations, and sequences [329].
In daily clinical practice, the systemic (peripheral) vascular resistance (SVR) is the most common parameter used to describe the actual afterload, and often, SVR is used synonymously with after- load.
SVR however, only reflects the non-pulsatile component of the peripheral load under steady state conditions [398]. It does not comprise the impact of wave reflections, arterial impedance, or ventricular properties. Each of these phenomena affect LV-afterload independently of peripheral vascular resistance or arterial pressure [376]. Ageing, hypertension, and aortic stiffening contribute considerably to the pulsatile component of the afterload, and thus, this component becomes more prominent under those conditions [399, 400]. Lang [401] showed in his investigation that the measurement of SVR substantially underestimates the change in afterload when LV afterload alone was decreased, increased, or remained unchanged, but with a simultaneous increase in contractility. These findings are not surprising, because from the peripheral pressure-flow relationship, the systemic peripheral resistance is not seen by the LV [378]. Nevertheless, SVR accounts for 90% of the resistance to ejection (arterial resistance is the dominant component of impedance load [402]) [403] and thus is justified as being the most commonly used parameter to clinically estimate afterload [222]. Furthermore, SVR may be very helpful in clarifying the diagnosis [13, 128, 222], particularly in hypotensive patients, and in heart failure syndromes, as shown by Cotter [13].
The fundamental pathophysiological alteration in acute heart failure syndromes is a substantially and inappropriately elevated afterload, with a markedly elevated systemic resistance/markedly increased LV outflow impedance, exerting a high (end-)systolic load on the LV during ventricular ejection [11, 19, 394]. This is referred to as afterload mismatch, defined by “a fall in SV due to inappropriately high afterload” [329, 394, 404]. In heart failure syndromes, the LV afterload becomes the decisive determinant of cardiac performance [11, 12, 14]. As early as 1977, Cohn and Franciosa published their impressive diagram showing the correlation between afterload and cardiac performance/cardiac output (SV) (see Fig. 1.6) [11].


Fig. 1.6
Relation between SV (SW) and outflow resistance/impedance (adapted from Cohn, J. N. and Franciosa, J. A. [11], with permission)
SV depends decisively on the magnitude of the afterload [3, 46, 93]. Furthermore, an elevated (after)load causes an increase in the LV filling pressure [405], and thus affects the already compromised diastolic properties of the heart, resulting in a further reduction of the LV filling rate [406, 407]. Afterload is inversely proportional to the stroke volume, SV ~1/afterload [394], and therefore an increase in afterload should result in a fall in SV and ejection fraction (EF) [378, 408]. However, in healthy hearts, despite an increase in wall tension due to the increased afterload, normal fibre shortening is accomplished by a compensatory increase in contractility [9, 93]. In the case of impaired LV function the increase in afterload is not tolerated, fibre length shortening is impaired, and a decrease in EF results [19].
Finally, remember the following [382, 383]:
afterload ↑ → LVESV ↑ [410] and SV ↓ [410] (in healthy persons SV may be maintained due to an increase in contractility),
Due to the law of LaPlace:
afterload ↓ → LVEDP ↓ → diastolic wall stress ↓ → O2–requirement ↓ [20, 410] → LVEDD ↓ [17, 18, 93, 411],
↓ aortic impedance (ventricular afterload) → ↓ systolic wall stress, and vice versa [412]
1.6 Contractility
1.6.1 Definition
Contractility is defined as the inherent capacity of the myocardium to contract independently of changes in pre- and afterload [413]. This capacity of Intrinsic Force of contraction is called Contractility or Inotropy [414, 415].
Braunwald writes, “Changes in cardiac performance independent of alterations in pre- and afterload are caused by ‘contractility’. It has to be separated from changes in the performance due to a change in loading conditions” [413].
The sympathetic tone plays an important role in the regulation of contractility. The positive inotropic effect of increased sympathetic tone enables the heart, without a change in diastolic filling (without a change in the preload), to eject a higher SV or to maintain SV in case of increased afterload or increased resistance to ejection [416]. Kumar [9] found in healthy volunteers that the increase in SV due to volume loading is predominantly caused by an increase in contractility and only in minor part by the Frank-Starling mechanism, hence confirming previous results [146, 147]. Due to the increase in ‘intrinsic’ contractility, the end-systolic volume will decrease [9].
1.6.2 Measurement and Quantification
It is very difficult to measure and to express contractility as a single, independent parameter. At the sarcomere level, contractility and load are interrelated; thus, they are not independent variables [417, 418]. Any parameter attempting to characterise ‘true’ contractility has to be independent of changes in pre- and afterload, LV-size and geometry and LV-pressure [419].
The rate of LV intraventricular pressure rise dp/dt, an index of the isovolumetric phase of the contraction [420], correlates well with the LV contractility [421]. The highest dp/dt, called dp/dtmax, throughout systole is expected to be proportional to the contractility [421]. Dp/dtmax, is sensitive of preload, but not of afterload because it is measured before the aortic valve opens [421].
Dp/dtmax shows reasonably good sensitivity to detect and express changes in the ‘true’ inotropic status (intrinsic contractility) [422, 423]. It is the most valuable parameter to measure and express inotropy [422–425] and is currently the gold standard in representing the ‘true’ (intrinsic) contractility [426].
The contractile conditions of the ventricle are influenced by intrinsic properties of the ventricle at end-systole, the chamber elastance (Ees). These contractile properties of the ventricle can be quantified by the relationship between end-systolic left ventricular pressure (LVESP) and the end-systolic left ventricular volume (LVESV) [388, 427].
The ventricular pressure-volume relationship at end-systole is linear (at least under physiological conditions [428]) and its slope, Ees, quantifies the ventricular (systolic) contractile properties [388, 428, 429] (read more in part 1.9.3 of this chapter) (Fig. 1.7).


Fig. 1.7
The diagram depicts the effect of an increase in true contractility: The slope of Ees becomes steeper, SV increases (SV2) and LVESV gets smaller (LVESV2). Thus the improvement in contractility is reflected by a larger SV ejected, leading to a smaller LVESV while the LVEDV remains unchanged
Ees is defined as LVESP divided by LVESV, thus


[430]
Ees is roughly load-independent [388], and Kass [431] found that over a wide range of load, Ees is a powerful index of true LV-contractility [432–435].
When describing the systolic properties of the heart, we must differentiate between indices referring to the ‘true’ contractility and to other parameters describing the systolic function of the heart muscle or the heart performance. The latter two are less independent than the other indices and haracterise the heart function in a more ‘global’ way. (For an overview see Baicu [368]).
LV systolic performance is characterised by the stroke work, taking into account that the heart has to generate pressure and flow (SV) [4, 93]:


Normal values: 58–104 gm−1 m2 [437]
The systolic performance is influenced by load and ventricular configuration [438]; thus, it is not the same as contractility. Hence, abnormal performance may be present although contractility is normal (i.e. in case of high afterload) and vice versa, performance may be normal although the contractility is impaired (i.e. sepsis, MR) [438].
Whilst cardiac work describes the transferral of energy from the cardiac contraction to the de- velopment of blood flow [128], cardiac power output (CPO) describes the amount of energy generated by the heart that the whole systemic vasculature receives at the level of the aortic root [128]. Thus, it characterises the recruitable reserve still available in case of acute failure or shock in order to maintain the perfusion of the vital organs and hence reflects the severity of the patient’s illness [132]. CPO has shown substantial prognostic power [128, 439] across the broad spectrum of acute heart failure syndromes and, in particular, in cardiogenic shock [128]. CPO is defined [128] as


and follows the physical rules of fluids. Reflecting the essential task of the heart (to generate pressure and flow) [3, 4] CPO is a measure of cardiac pumping by coupling both pressure and flow domains [440].
Furthermore, CPO and its index, CPI, have shown superiority in determining the exact diagnosis of the actual heart failure syndrome compared to CI, BP, PCWP and their combination [13, 128]. Whilst the traditional haemodynamic measures and their presumed target values used in treatment protocols have been misleading [441], they have also failed to show any relevant effect when therapy was titrated upon reaching these values [442].
CPO appears to be a better parameter than CPI for predicting outcome. Adjustment of CPO for body size, yielding CPI, showed a weaker association with mortality [443, 444]. A CPO ≤ 0.53 most accurately predicts a high likelihood of in-hospital mortality [128, 439].
Conventionally SVI and SWI were used as powerful predictors of short term mortality in cardiogenic shock complicating AMI [445], but the use of CPO is now thought preferable.
The LV systolic function of the heart can be described in a number of ways but, ejection fraction (EF, %) is still the most frequently used parameter. EF is determined by the interaction of arterial and ventricular properties and is dependent on the afterload, and thus it is not exclusively governed by the LV [347, 368, 446].
![$$ \mathbf{EF}\%=\left[\left(\mathbf{LVEDV}-\mathbf{LVESV}\right)/\mathbf{LVEDV}\right]\times \mathbf{100}; $$](https://i0.wp.com/thoracickey.com/wp-content/uploads/2017/09/A183660_2_En_1_Chapter_Equt.gif?w=960)

![$$ \mathbf{EF}\%=\left[\left(\mathbf{LVEDV}-\mathbf{LVESV}\right)/\mathbf{LVEDV}\right]\times \mathbf{100}; $$](https://i0.wp.com/thoracickey.com/wp-content/uploads/2017/09/A183660_2_En_1_Chapter_Equt.gif?w=960)

However,


As such, EF may, by all means, be considered as a good coupling parameter, describing fundamental aspects of ventriculo-arterial coupling [449, 450] rather than contractility.
EF is thus far from being an ideal parameter to assess contractility. EF depends on afterload as well as on preload and heart volume or mass [394, 423, 451, 452].
EF will fail to report:
excess afterload (EF reduced although the contractility is normal) [453],
in case of augmented preload (i.e. MR), EF will overestimate the systolic function, missing myocardial dysfunction [454, 455],
in concentric LV-H, EF measurement signals normal systolic function, although substantial dysfunction may be present [456].
Despite its shortcomings, Braunwald [438] and Gillebert [463] state that EF is the best parameter to describe overall contractility in comparison to all others currently in use.
‘True’ LV-contractility is best expressed by:
Ees <1 mmHg/mL is found in dilated and failing hearts [464], in case of hypertrophy there will be a significant increase—up to 4 mmHg/mL [465].
It has to be stressed that CI is not an index of contractility, but rather a measure of cardiovascular flow: CI is affected by contractility, vascular stiffness and resistance, intravascular volume and filling pressures [128]. Furthermore, there is no normal CO/CI, since metabolic demands can vary widely [117].
1.6.3 Inotropic Medications
Medications able to increase the myocardial contractility are called inotropes. In recent years the administration of inotropic drugs has been overshadowed by clear and growing evidence of adverse events and increased mortality [143, 466–470], particularly when given in patients with reasonably preserved left ventricular function (EF > 40%) [471, 472]. Conners [473] and Sandham [474] found a significantly increased mortality when clinically stable patients were treated with conventional inotropic agents secondary to numerically low cardiac output. Only patients who absolutely require inotropic support secondary to low output as result of severely impaired contractility and who are resistant to other treatments should be treated by such drugs [462, 475].
The European Society of Cardiology (ESC) recommends inotropic agents in heart failure syndromes if the illness has deteriorated to become life-threatening and the situation has become critically dependent on the haemodynamics: “Inotropic agents are indicated in the presence of peripheral hypoperfusion with or without congestion or pulmonary oedema refractory to diuretics and vasodilators at optimal dosages” [462].
1.7 Heart Rate and Contractility
At the end of the 19th century Bowditch published his observation that the force of heart con- traction increases—up to a limit—with an increase in heart rate [414].
The peak isometric force increases with increasing heart rate [414, 476]. This is due to the fact that calcium will accumulate within the myocytes when diastole shortens [477] (which happens with increasing heart rate). In the case of a compromised or failing heart this effect is attenuated, or even the opposite may happen—with increasing heart rate the force of contraction will decrease [476, 478]. When the tachycardia exceeds 130/min, the severity of myocardial impairment correlates with the extent of tachycardia [479]. Furthermore, tachycardia will always precede a fall in BP [293].
Thus, in the case of tachycardia in a compromised heart the reduction in heart rate will increase the cardiac contraction and hence SV (MAP and organ perfusion):


[480]
In heart failure patients developing or suffering from atrial fibrillation, a heart rate of 100–110/min is acceptable [481].
1.8 Diastolic Ventricular Interaction/Interdependence (DVI)
1.8.1 Definition
The right and the left ventricle are anatomically and functionally closely interrelated, since they share the interventricular septum (IVS), the pericardium, and (by their continuity) parts of myocardial fibres [482]. Ventricular interdependence characterizes the “response of one ventricle to the changes in pressure and volume of the other” state Elzinga et al. [483]. However, the interactions include even more features as they refer to the changes in size (volume), shape, pressure, and (concomitantly) compliance of one ventricle due to direct, predominant mechanical (independent of neurohormonal and circulatory effects ), impact on the other [482], and further to a systolic contribution of the LV to RV contractile performance [393, 484]. Accordingly, diastolic ventricular interaction largely refers to the competition of the two ventricles for space within the non-distensible pericardial sack, namely when RV dilates, and systolic ventricular interaction applies to the contribution of LV to RV systolic performance (read more about this issue in Chap. 4) [393]. The impact may be even dramatic in case of acute changes in RV size and pressure [24, 41, 47, 485].
These diastolic interactions are mediated via the shared structures of the two ventricles, the interventricular septum and the pericardium. The pericardium has constraining effects on ventricular filling due to its poor distensibility and its pressure transmitting effects [47, 486]. The interaction mediated by the septum and the pericardium is called ‘direct’ interaction, compared to the so called ‘series’ interaction which refers simply to the physical relation between the two ventricles and their outputs: The two ventricles are coupled in a row, one after the other and thus their output necessarily has to be equal over time [31, 41].
1.8.2 Septum and Trans-septal Pressure
The shape of the septum, under physiological conditions, is concave when viewed from the LV side. There is no difference during systole and diastole, due to the fact that the LVEDP always remains higher than the RVEDP and increases proportionately during systole [41]. Kingma established proof that the position of the septum is determined by the end-diastolic pressure gradient between LV and RV [487]:


[487].
In disease, the position of the septum can change markedly due to changes in the pressure gradient, which will alter the end-diastolic volumes substantially [47, 48, 119, 487, 488]. In acute RV pressure or volume overload Kingma showed that the interventricular septum becomes flattened or even convex at end-diastole due to RV dilatation and raised RVEDP, diminishing the transseptal pressure gradient and pushing the septum towards the left ventricle [487]. Numerous publications confirm the change in the septum position in different diseases such as acute and chronic pulmonary hypertension [47, 48, 489], congestive heart failure [23, 24], and mechanical ventilation [119].
This leftward shift of the septum contributes significantly to the reduction in LV-filling; thus, total LV-volume and end-diastolic volume are reduced and the SV will fall as a consequence.
1.8.3 Pericardium
All cardiac chambers (except the posterior part of the LA where the pulmonary veins enter) are enclosed by the pericardium. It works as a tight, unyielding band around the minor axis of the heart, fixing the cross sectional area of the heart and causing direct ventricular interaction [491].
Thus, an increase in the cross-sectional area of one ventricle, e.g. due to volume loading or enlargement, necessarily reduces the area of the opposite ventricle with less filling potential, causing an increase in the pericardial pressure, and altering the transmural pressure [23, 491]. The total cardiac volume remains unchanged [488, 490].
Increasing pressures in the pericardial space will exert a progressive restraining effect on ventricular filling, termed pericardial constraint [488]. When the pericardium becomes stretched due to enlargement of the ventricles, such as in chronic heart failure or due to volume loading, the filling—in particular the left ventricular filling—becomes significantly restrained [23, 127]. With further stretch the pericardium is even less distensible [486] and, especially in cases of acute change, the pericardium, with its constraining effect, plays a key role in loading conditions [85, 492–494]. Under those conditions the pericardial pressure (PP) will increase progressively and will significantly constrain the filling. PP rises in an exponential manner [491] and once the pericardium becomes ‘overstretched’, an exponential increase in LVEDP is seen [83, 495].
Raised intra-thoracic pressure, e.g. due to raised intra-abdominal pressure, chest infection, etc., will affect, secondary to an increased constraint on the thin walled RV, the RVEDP more than the LVEDP (rise in RVEDP > rise in LVEDP) [47, 48]. Hence, the transmural LVEDP (= LVEDP − RAP/CVP) will decrease with less LVEDV and less LV end-diastolic fibre stretch, and a reduced SV will result.
Ventricular interaction due to pericardial constraint is diminished as long as the PP is <5 mmHg [126]; when exceeding 9–10 mmHg the pericardium will exert a significant constraint on ventricular filling [63, 127]. Furthermore, when intraventricular LVEDP exceeds 10(12)–15 mmHg, the LVEDP-LVEDV relation becomes much steeper and the pericardium limits further increases in LV volume [83, 133, 495].
1.8.4 Pulmonary Hypertension and the Risk of DVI
In pulmonary hypertension fluid administration is shown to increase RVEDP more than LVEDP [47, 48]. The concomitant (along with RVEDP) increase in pericardial pressure will exceed the rise of the LVEDP (due to a higher increase of RVEDP compared with LVEDP), thus transmural LVEDP and therefore LV-preload will be reduced due to pericardial constraint [23, 51] (Fig. 1.8).
Fluid administration in pulmonary hypertension
Get Clinical Tree app for offline access
1.8.5 Acutely Exacerbated Chronic Congestive (Left-Sided or Biventricular) Heart Failure
An acute exacerbation of chronic congestive heart failure is often crucial in the disease’s course and may be the final point in a critical illness [496, 497]. In this situation, DVI may have a substantial impact on the haemodynamics and has to be taken into the therapeutic considerations [47, 48, 498, 499].
A sudden rise in RV-afterload/increase in RV-outflow impedance, e.g. pulmonary embolism, PE, and/or a loss in contractility, e.g. due to acute RV myocardial infarction [500, 501], will always induce RV dilatation [502, 503], a fall in RV-EF [502, 503] and a substantial increase in RVEDP [69, 382, 504]. This implies a considerable rise in PP and a leftward shift of the septum, which compromises LV filling [23, 39, 42, 44, 54, 56, 63, 487, 488, 490].
![$$ \begin{array}{c}\mathbf{Acute}\kern0.5em \mathbf{RV}-\mathbf{outflow}\ \mathbf{impedance}/\mathbf{RV}-\mathbf{afterload}\\ {}\downarrow \\ {}\mathrm{RV}-\mathrm{dilatation}\left(\mathrm{RVEDD}\ \right),\kern0.5em \mathrm{RV}\mathrm{EDP},\ \mathrm{and}\downarrow \mathrm{RV}-\mathrm{EF}\\ {}\kern12.75em \downarrow \kern0.5em \mathbf{DVI}\left[47,\ 48,\ 498,\ 499\right]\\ {}\downarrow \mathrm{transmural}\ \mathrm{LVEDP}\to\ \downarrow \mathrm{LV}-\mathrm{SV}\ \left(\mathrm{LV}-\mathrm{SW}\right)\ \left[\ 23,\ 39,\ 42\right]/\downarrow \kern0.5em \mathbf{blood}\ \mathbf{pressure}.\end{array} $$](https://i0.wp.com/thoracickey.com/wp-content/uploads/2017/09/A183660_2_En_1_Chapter_Equz.gif?w=960)
![$$ \begin{array}{c}\mathbf{Acute}\kern0.5em \mathbf{RV}-\mathbf{outflow}\ \mathbf{impedance}/\mathbf{RV}-\mathbf{afterload}\\ {}\downarrow \\ {}\mathrm{RV}-\mathrm{dilatation}\left(\mathrm{RVEDD}\ \right),\kern0.5em \mathrm{RV}\mathrm{EDP},\ \mathrm{and}\downarrow \mathrm{RV}-\mathrm{EF}\\ {}\kern12.75em \downarrow \kern0.5em \mathbf{DVI}\left[47,\ 48,\ 498,\ 499\right]\\ {}\downarrow \mathrm{transmural}\ \mathrm{LVEDP}\to\ \downarrow \mathrm{LV}-\mathrm{SV}\ \left(\mathrm{LV}-\mathrm{SW}\right)\ \left[\ 23,\ 39,\ 42\right]/\downarrow \kern0.5em \mathbf{blood}\ \mathbf{pressure}.\end{array} $$](https://i0.wp.com/thoracickey.com/wp-content/uploads/2017/09/A183660_2_En_1_Chapter_Equz.gif?w=960)
Atherton [24] showed that, in patients with chronic congestive heart failure and high LVEDP (causing pulmonary venous hypertension), LV-filling was markedly impeded due to direct diastolic ventricular interaction via the septum and from the stretched pericardium (pericardial constraint): Volume unloading resulted, as expected, in reduction of the RVEDV, but LVEDV “paradoxically” increased (see Fig. 1.9).
In nearly 50% of all patients suffering from congestive HF, pericardial constraint plays a marked role [24] and unloading leads to an improvement in cardiac performance. Even if there is less pericardial constraint present, as in the other 50% of patients studied by Atherton, the reduction in LVEDV secondary to volume unloading did not significantly compromise the haemodynamic situation.
These results are consistent with the findings by Dupuis, who showed that a reduction in PCWP in patients with congestive HF resulted in an increased SV and SW even though LVEDP fell [49]. Stevenson established in 1986 that volume unloading in patients with severe congestive heart failure and high filling pressures showed clear beneficial results, with an improvement in clinical short and long term outcome [67].
Moore explored the underlying pathophysiological mechanisms and established our current therapeutic approach [23]. In patients with congestive HF, and thus secondary pulmonary hypertension, direct diastolic ventricular interaction plays a substantial role in the LV-dysfunction responsible for the reduced LV-SV. The common approach of administering volume to a patient with low blood pressure will, in acutely decompensated chronic heart failure, worsen the haemodynamic and clinical situation [23]. Volume unloading will stabilise the situation (Fig. 1.9).


Fig. 1.9
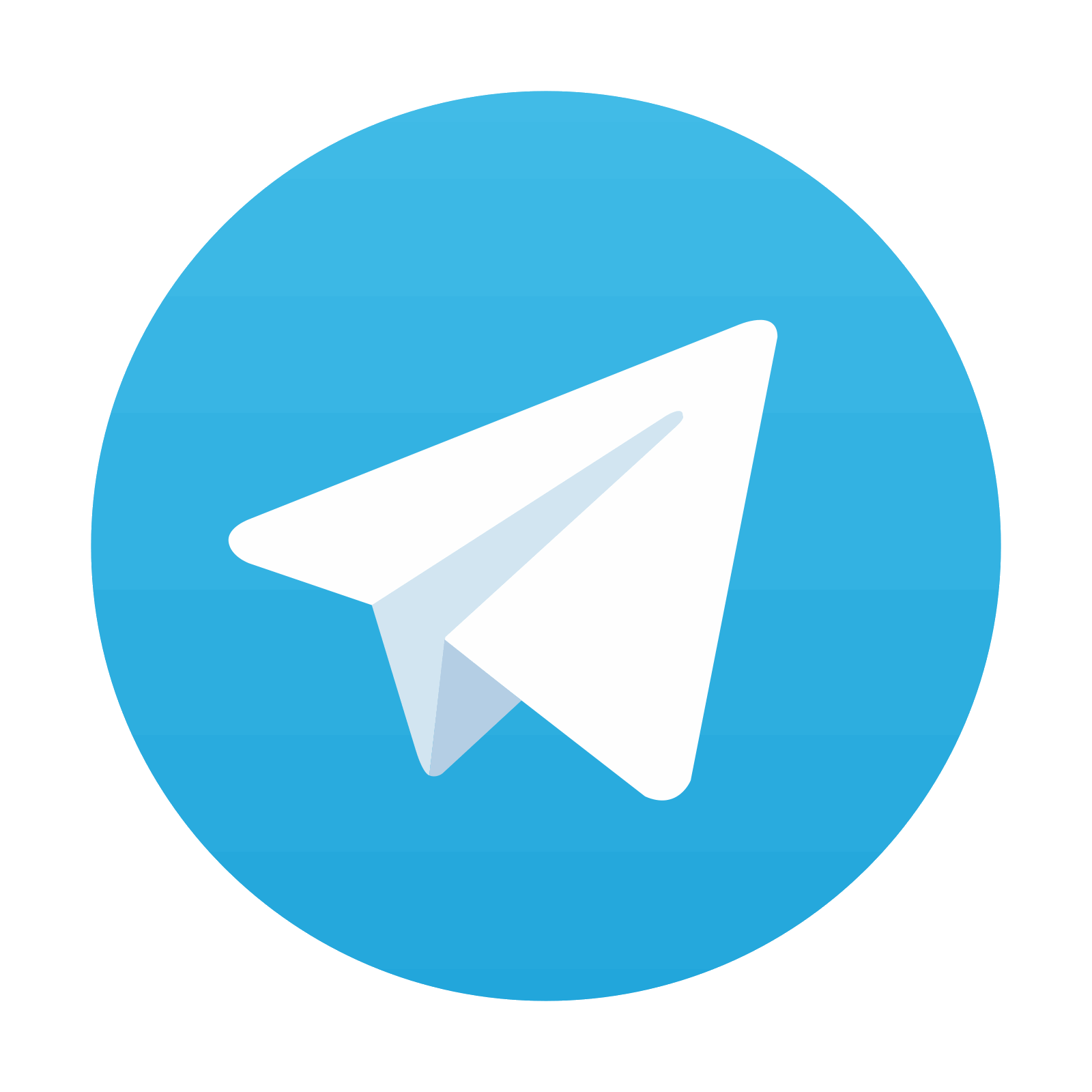
Diagram to show the position of the interventricular septum in different loading conditions due to DVI effects in patients with bi-ventricular heart failure
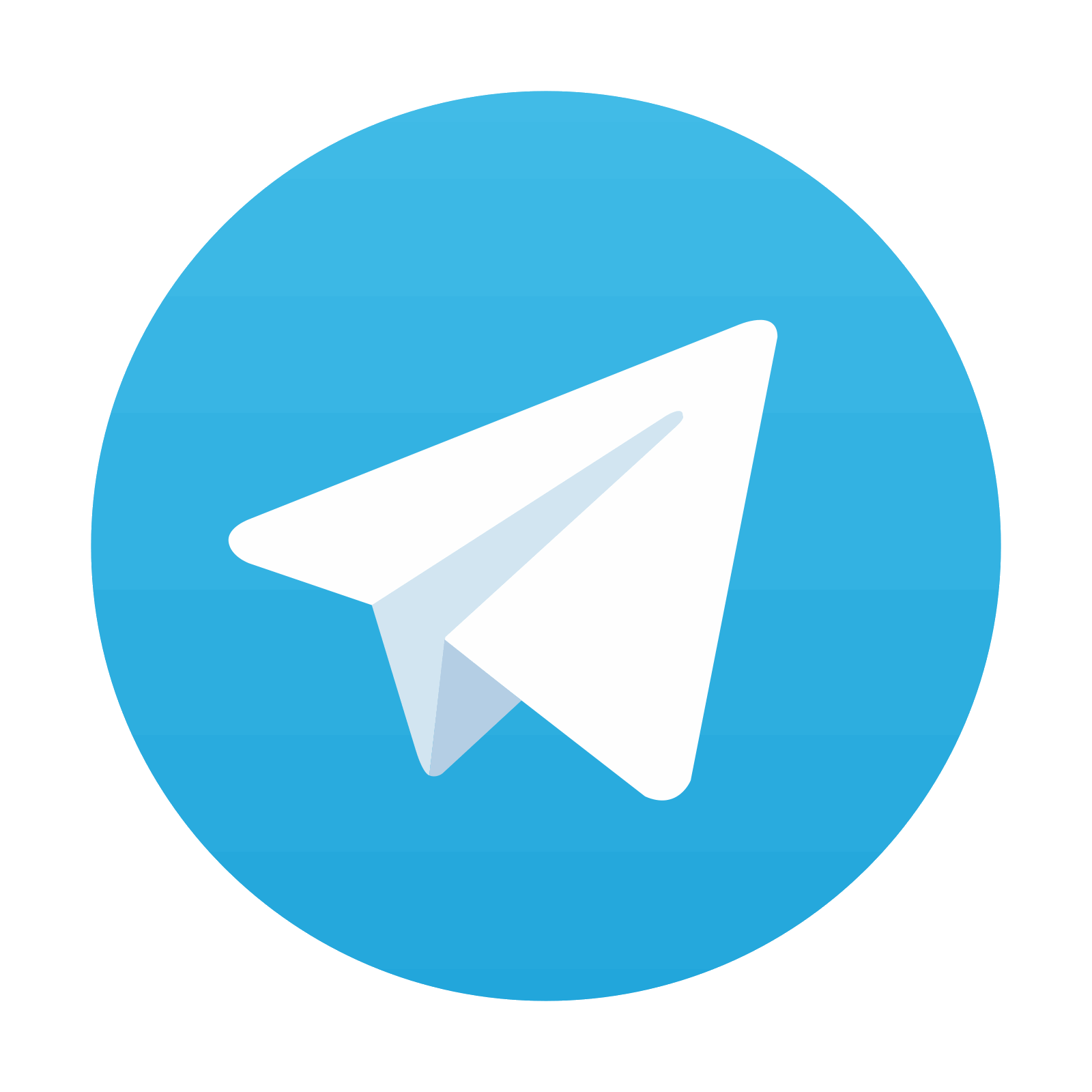
Stay updated, free articles. Join our Telegram channel
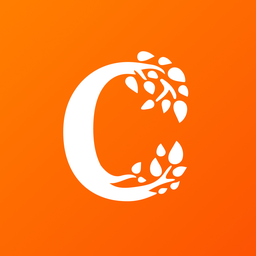
Full access? Get Clinical Tree
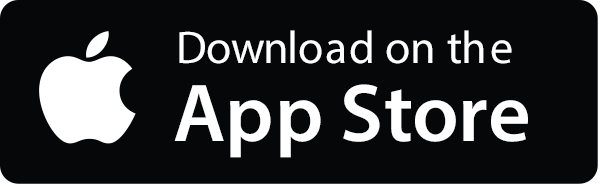
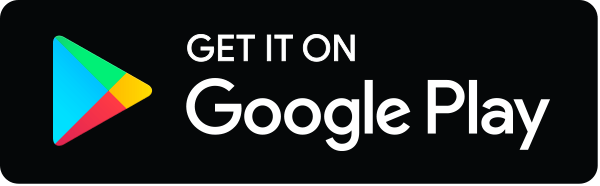
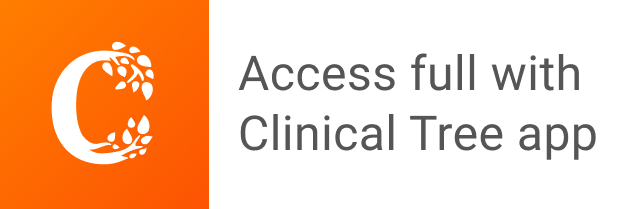