We take for granted the relative ease that we are now able to measure the rate at which the heart pumps blood, better known as the cardiac output. It is a central parameter for clinically important measurements such as the determination of the status of the patient in known or suspected shock and the calculation of valve area and vascular resistances (pulmonary and systemic vascular resistance). Because of the importance of cardiac output measurement, it is necessary to understand the methods and limitations of its calculation in the cardiac catheterization laboratory.
Adolph Fick described the theoretical basis for cardiac output determination in man in 1870, but more than 60 years elapsed before clinical application became possible. André Cournand described the first set of cardiac output measurements in humans in 1945 and noted:
The practical difficulty preventing the ready application of this (i.e., Fick’s) principle in human subjects has been that of obtaining reliable samples of average or mixed venous blood. With the development of the technique of catheterization of the right heart, this difficulty has been largely overcome, and the experience of the last 3 years has led to a procedure for determining cardiac output in man which can be used in almost all forms of disease or injury, with safety and without discomfort to the patient beyond that attendant upon the insertion of a needle in the femoral artery, and cutting down on a median basilic vein both under novocaine anesthesia.
Currently the determination of cardiac output and the calculation of the magnitude of an intracardiac shunt are routine and integral parts of cardiac catheterization. The performance of these measurements, their limitations, and their role in modern catheterization are discussed here.
Measurement of Cardiac Output
Cardiac output can be determined by one of several techniques: Fick (and “assumed Fick”) methods, indicator-dilution method, thermodilution method, and angiography. Clinical catheterization laboratories and intensive care units rely primarily on the Fick and thermodilution methods. Cardiac output is expressed in liters/minute and is often corrected for patient size by dividing by the body surface area, which converts the measurement to the cardiac index in units of liters/minute/meter 2 . The normal cardiac output at rest is 5 to 8 L/min, and the normal value for resting cardiac index is >2.4 L/min/m 2 . With exercise, cardiac output increases substantially; elite athletes achieve cardiac outputs in excess of 30 L/min.
Fick Method for Cardiac Output Determination
Adolph Fick described the theoretical basis for cardiac output determination nearly 150 years ago. Fick’s principle states that the total uptake or release of a substance by an organ is the product of the blood flow to the organ and the arteriovenous concentration difference of that substance. Using the lungs as the organ and oxygen as the substance, blood flow to the lungs can be calculated by Fick’s relationship, as follows:
Pulmonarybloodflow=OxygenconsumptionArteriovenousoxygendifference
In the absence of a shunt and because pulmonary blood flow equals systemic blood flow, the relationship can be used to calculate systemic cardiac output. The arteriovenous oxygen difference is the difference in oxygen content in the blood across the pulmonary circulation and is calculated as the arterial oxygen content minus the mixed venous oxygen content. Oxygen content is determined as the product of blood oxygen saturation, the hemoglobin concentration (in grams per deciliter), and the amount of oxygen carried per gram of hemoglobin (1.36 mL oxygen per gram of hemoglobin). This value is multiplied by 10 to correct the units (grams/deciliter to grams/liter). Thus Fick’s formula for determining cardiac output is
Cardiacoutput=Oxygenconsumption(Arterialsaturation-Mixedvenoussaturation)×(Hgb)×13.6
Fick’s principle states that the arteriovenous oxygen (AV-O 2 ) difference is inversely proportional to the cardiac output. A normal AV-O 2 difference is about 20 to 50 mL/L. At low cardiac outputs, there is greater extraction of oxygen from the tissues, and the mixed venous saturation is low, resulting in a high AV-O 2 difference. In high-output states, diminished extraction leads to high mixed venous saturations and low AV-O 2 difference. Note that the mixed venous saturation alone is often used in clinical practices as a crude but rapid estimation of the cardiac output state, with a low mixed venous saturation indicating a low cardiac output and a high saturation indicating a high output (in the absence of a shunt). Furthermore, when cardiac output is low, the AV-O 2 difference is large, making it easier to measure and therefore making it a more accurate method at low cardiac outputs.
The important variables measured in the cardiac catheterization laboratory are the AV-O 2 content difference and the oxygen consumption. The AV-O 2 content difference is easily and accurately measured by simultaneously obtaining systemic arterial and mixed venous blood samples. Ideally, the arterial blood sample would be obtained from a pulmonary vein; however, in the absence of a right-to-left intracardiac shunt, the central aortic, femoral, or radial artery oxygen content closely approximates pulmonary vein oxygen content. In the absence of intracardiac shunting, the pulmonary artery is the most reliable site for obtaining mixed venous blood as opposed to other proximal sites such as vena cava, right atrium, or right ventricle, where there may be significant variation in blood oxygen content within the chamber. These blood samples are analyzed for the percentage of oxygen saturation. To determine oxygen content, the AV-O 2 difference is multiplied by the measured hemoglobin, which is then multiplied by the oxygen-binding coefficient (1.36 mL O 2 /g of hemoglobin). The product is multiplied by 10 to convert units from grams/deciliter to grams/liter.
The other important measurement used to calculate the cardiac output by Fick’s method, and a potential source of error, is oxygen consumption. At steady state, oxygen consumption is the rate at which oxygen is taken up by the blood from the lungs and should ideally be measured directly in the catheterization laboratory for each patient. Historically, oxygen consumption was directly measured using a Douglas Bag over a number of minutes, but this direct collection of expired air requires a steady-state environment, cooperation of the patient, and technical personnel who are familiar and experienced with the equipment and methodology. This method is time-consuming and cumbersome and has been largely abandoned in favor of the “assumed Fick” method, which is based on equations developed by LaFarge and Miettinen, as shown in Table 3.1 . While relatively reliable and in widespread use today, the equations were originally developed using regression analysis of data from younger congenital heart disease patients with a mean age of 12 years and subsequently validated in broader patient cohorts. However, the observation, that oxygen consumption is known to vary during the cardiac catheterization procedure and may be significantly different from “assumed” values, has resulted in increasing interest in using more modern techniques to directly measure oxygen consumption in each patient concurrently with measurements of oxygen saturations. Cardiac output calculated by direct continuous oxygen consumption measurements can differ significantly from the calculated “assumed” values. Various modern, portable commercial systems can continuously determine oxygen consumption by measuring breath-by-breath oxygen levels using a facemask and can be used in the cardiac catheterization environment.
Authors, Year | Name | Equation | Developmental Cohort Summary |
---|---|---|---|
Dehmer, Firth, and Hills, 1970 | Dehmer | VO 2 = 125 × BSA a | n = 108, mean age = 49 yrs (SD not provided), % male = 64, % cardiomyopathy = 9.0 |
LaFarge and Miettinen, 1970 | LaFarge | VO 2 = 138.1 − (11.49 × log age) + (0.378 × HR) × BSA a for males; VC 2 = 138.1 − (17.04 × log age) + (0.378 × HR) × BSA for females | n = 879, mean age not provided, between 3 and 40 yrs, % male = 59, % cardiomyopathy not provided |
Bergstra, van Dijk, Hillege, Lie and Mook, 1995 | Bergstra | VO 2 = 157.3 × BSA a + 10 − (10.5 × log age) + 4.8 for males; VO 2 = 157.3 × BSA a − (10.5 × log age) + 4.8 for females | n = 250, mean age 34.6 ± 22.7 yrs, % male = 57, % cardiomyopathy not provided |
a BSA calculated using the formula of Dubois 16 with BSA (m 2 ) = 0.007184 × weight (kg) 0.425 × height (cm) 0.725 .
Because of its simplicity, most catheterization laboratories and commercial hemodynamic systems still use an “assumed” value for oxygen consumption based on the patient’s age, gender, and body surface area. The assumed value is typically 125 mL/min/m 2 for average individuals and 110 mL/min/m 2 for elderly patients. However, the obvious differences among patients who undergo cardiac catheterization introduce errors in cardiac output determinations as oxygen consumption varies significantly among adults at the time of cardiac catheterization, which introduces discrepancies between direct measurement of oxygen consumption and the assumed values, particularly in patients with hypoperfusion or cardiogenic shock.
Although the use of “assumed” rather than directly measured O 2 consumption is the major source of error in the Fick method, additional errors may be introduced because of improper collection of the mixed venous or arterial blood samples. Ideally, these blood samples should be collected simultaneously in a steady-state environment. Errors may be present if, for instance, arterial blood is sampled early in the case, and mixed venous blood is sampled at a later time in the procedure, when the patient may become oversedated, thereby causing the patient to hypoventilate and resulting in the lowering of the mixed venous oxygen content, falsely implying a lower cardiac output. In addition, care should be taken to ensure that the mixed venous sample is obtained from the pulmonary artery and that the pulmonary artery catheter has not migrated to a “wedge” position that will falsely elevate the oxygen saturation. When the Fick method is performed correctly, the total error in the determination of the cardiac output is about 10%. Fig. 3.1 shows an example of Fick’s method for cardiac output calculation.

Indicator-Dilution Method for Cardiac Output Determination
Also based on Fick’s principle, the cardiac output can be derived by studying the flow characteristics of an indicator substance. On injection of a substance into the circulation, the rate at which the indicator appears and disappears from a downstream point correlates directly with the cardiac output. For example, if the cardiac output is high, the indicator will rapidly appear and quickly wash out; if the cardiac output is low, the indicator will require a longer time to achieve its maximal concentration and a longer time to wash out. Therefore the area under the time-concentration curve is related inversely to the cardiac output. The Stewart-Hamilton formula for the calculation of cardiac output takes into account the mean concentration of the indicator during the first passage and the duration of the extrapolated curve:
Cardiacoutput(L/min)=Amountofindicatorinmg×60 s/minConcentrationofindicator(mg/mL)×curveduration(s)
Performance of this technique involves injection of a bolus of indicator into a systemic vein, the pulmonary artery, or the left atrium. The indicator mixes with the blood, and its concentration is measured continuously as a function of time at a sampling site (generally, the aorta, radial, or femoral artery). Numerous indicators have been used, with indocyanine green dye representing the most commonly used indicator. The normal curve generated with this method has an initial rapid upstroke followed by a slower downstroke and continued appearance due to the recirculation of the tracer. This recirculation creates some uncertainty at the tail end of the curve, and extrapolation of the curve is necessary to correct this distortion. The downslope of the primary curve is projected to the baseline to exclude indicator recirculation. Planimetry of the area under the curve yields the cardiac output.
This method has several limitations. Indocyanine green dye is unstable over time and can be affected by light. The indicator must mix well with blood before reaching the distal sampling site, and it must have an exponential decay over time so that extrapolation of the time/concentration curve can be accurately performed. This technique is not accurate in the presence of irregular rhythms, valvular regurgitation, or intracardiac shunts. Importantly, this method is inaccurate in low output states in which the wash out of the indicator is prolonged. In these cases, recirculation of the indicator begins well before an adequate decline in the indicator curve occurs, distorting the downslope of the curve before it reaches baseline and preventing correction for recirculation. When indicator-dilution measurements are compared with the Fick method, the disparity between the two measurements is increased in patients with low cardiac output and those with aortic and mitral regurgitation. The disparity between the Fick method and indicator-dilution measurements is greater than the disparity between Fick and thermodilution measurements. This technique has essentially been abandoned by clinicians and is primarily of historical interest.
Thermodilution Method for Cardiac Output Determination
Fegler described the thermodilution method in 1954. This method is the easiest to perform and the most widely used method to measure cardiac output in catheterization laboratories and critical care units.
The thermodilution technique is a variation of the indicator-dilution technique, using blood temperature as the indicator. Saline at a known temperature is injected into the right atrium from the proximal port of a Swan-Ganz catheter. The saline mixes with the blood and lowers its temperature. The temperature of blood is measured in the pulmonary artery by a thermistor mounted on the distal tip of the catheter. The thermistor is a variable resistor in which the resistance is proportional to the temperature. As the resistance changes, a change in voltage occurs. The measured change in voltage over time generates a temperature curve that is related to the cardiac output. Similar to the indicator-dilution method, cardiac output is inversely related to the area under the time-temperature curve. If the area under the curve is small, this means the temperature equilibrates rapidly with the ambient body temperature, indicating a high cardiac output. Conversely, if the area under the curve is large, it takes longer for the blood temperature to reach ambient body temperature, implying low cardiac output. The cardiac output is calculated using an equation that takes into account the temperature and specific gravity of the injectate and blood and the injectate volume.
The thermodilution method is preferable to the indicator-dilution method because right-sided injection and right-sided sampling of the cold saline yield a curve that is less subject to recirculation-induced distortion than right-sided injection and left-sided sampling of indocyanine dye. Examples of a modern, computer-based system to measure cardiac output using the thermodilution technique are shown in Figs. 3.2 and 3.3 . Note that the temperature plotted on the y- axis decreases as the saline bolus passes the thermistor on the pulmonary artery catheter. The curve of a patient with normal output shows a rapid drop in temperature, whereas patients with low output have a much slower drop in temperature.
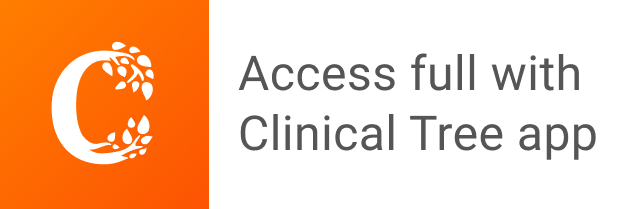