Fig. 12.1
Cardiac magnetic resonance imaging (MRI) four-chamber (A 1 –C 1 ) and short-axis cine images (A 2 –C 2 ) obtained in a patient with pulmonary arterial hypertension (PAH) over time. The course from right ventricular (RV) structural compensation to end-stage RV failure is demonstrated. This patient was diagnosed with an elevated mean pulmonary artery pressure (PAP) of 45 mmHg and a cardiac output (CO) of 4.1 L/min. At baseline, the RV shows a concentric RV remodeling pattern with increased RV mass, a crescent RV shape, small amounts of dilatation, modest RV function (right ventricular ejection fraction (RVEF): 39 %), and preserved left ventricular (LV) function (left ventricular ejection fraction (LVEF): 62 %) (A 1 , A 2 ). During 7 years of follow-up, PAP was unchanged and CO remained relatively stable. However, the RV remodeling pattern has changed (B, C). RV end-diastolic volume (RVEDV) showed a progressive increase from 140 to 449 mL. Smaller increases in RV mass were observed. Furthermore, the RV developed a spherical shape, apical ballooning, bulging of the interventricular septum into the LV (dark arrows), an enlarged right atrium, tricuspid insufficiency, and pericardial effusion (white arrows). In addition, RVEF and LVEF showed a progressive decline to 8 % and 29 % respectively. PAP (m/s/d) pulmonary artery pressure (mean/systole/diastole), SV stroke volume
The combined assessment of multiple RV imaging parameters provides insights into RV remodeling. According to a basic physiological principle of Guyton and Hall, the heart primarily functions as an on-demand pump to maintain CO in order to fulfill the needs of sufficient oxygen delivery to the body [13]. In the setting of chronic RV pressure overload, the RV compensates enduringly (i.e., by Frank–Starling mechanism [see also Chap. 2], RV dilatation, RV hypertrophy, tachycardia, changes in contractility) in order to sustain the CO. However, in the long run RV compensation mechanisms may get exhausted resulting in disturbed intrinsic RV structure and ultimately RV dysfunction and failure. Importantly, imaging of the RV might be of clinical relevance since it is likely that early changes in the RV structure could predict an ultimate RV functional deterioration (Fig. 12.1).
Based on the combined MRI assessment of RV mass, shape and volumes and by measures of RV pressure, ventricular wall stress can be estimated using the law of Laplace (i.e., wall stress = intraluminal pressure times chamber internal radius, divided by wall thickness) [14]. According to Laplace, RV hypertrophy is considered part of the adaptive remodeling response to increase RV pumping effectiveness by unloading of the individual muscle fibers and lowering ventricular wall stress. In contrast, an increased RV mass measured by cardiac MRI is associated with an increased mortality in scleroderma-associated PAH patients [15] and might have some prognostic relevance in idiopathic PAH [16]. These still ambiguous findings might be explained by several aspects. First, although RV mass in patients with PAH measured by MRI can be more than 2.5 times increased when compared to normal reference values [17], the RV often faces at rest a fourfold increase in pulmonary artery pressures indicating that the amount of RV hypertrophy may or may not be insufficient to lower the wall stress and protect RV function. This is in keeping with several MRI studies showing that, in PAH patients RV mass and pulmonary pressures are only modestly related [15, 18, 19]. Furthermore, it has been observed that patients with PAH associated with the Eisenmenger syndrome showed a higher amount of RV hypertrophy despite a similar elevation in pulmonary pressure compared to idiopathic PAH patients; in those patients with RVH was associated with better RV function and survival [20, 21]. However, in RV autopsy tissue of PAH patients and in rat models with pulmonary hypertension (PH) it has been found that an increase in RV mass is not necessarily accompanied by a similar increase in the number of contractile elements [22]. Second, using MRI with gadolinium contrast it has been demonstrated that degenerative changes occur in the hypertrophic RV of PAH patients. It was found that delayed contrast enhancement (DCE) appears as a unique pattern at the IVS insertion points and might be a reflection of focal fibrosis [23–26]. The extent of DCE was most strongly correlated with increased RV mass, volumes, and pulmonary pressures (i.e., RV wall stress) [23, 24]. Third, RV hypertrophy is associated with maladaptive changes in RV metabolism and blood flow (see below).
During chronic pressure overload, the RV phenotype changes from a more concentric (Fig. 12.1a) towards an eccentric remodeling pattern (Fig. 12.1b, c). The RV shape becomes more like the LV. In contrast to the crescent shape and bellows-like contraction pattern of the normal RV wall, a spherical RV shape in PAH might tolerate high pressure without generating high wall stress due to a decreased RV wall curvature radius of end-systole [27]. In addition, the RV dilates and it has been found by MRI that increased RV end-diastolic volume at baseline and progressive dilatation during follow-up were among the strongest predictors of mortality in PAH [16]. Although RV dilatation might be beneficial at first resulting in increased preload via the Frank–Starling mechanism to increase contractility and sustain CO, this compensatory mechanism eventually fails and results in a maladaptive course of remodeling with increased wall stress, disturbed RV function, and ultimately depressed RV output.
RV Contractility
Load-independent RV contractility can be assessed by right heart catheterization according to the single-beat method [28–32]. Noninvasive MRI approaches have also been developed to estimate RV contractility [33, 34]. In PAH patients, RV contractility is increased compared to controls. However, paradoxically global RV systolic function (e.g. determined by RV ejection fraction and stroke volume) is significantly decreased in PAH. This paradox is explained by the fact that in PAH, RV hypercontractility augmentation is insufficient for the degree of pressure overload, a phenomenon which has been described as ventriculo-arterial uncoupling [28, 29, 33, 34] (see also Chap. 2). In addition to a systolic impairment, it was recently demonstrated that RV diastolic stiffness is increased in PAH patients and this was related to disease severity [28].
RV Function
Imaging parameters describing global systolic RV function are all load dependent and therefore reflect RV-arterial coupling rather than intrinsic RV contractility. In fact, global RV systolic function is determined by multiple factors: preload, afterload, contractility, ventricular synchrony, valvular regurgitation, and shunt fraction [3, 35]. Low stroke volume and low RV ejection fraction at baseline and a further decrease during follow-up were associated with worse outcome [4, 16, 36]. Furthermore, stroke volume determined by MRI has been validated as the imaging parameter to monitor therapeutic effects [37]. In addition, after current medical therapies, it has been found that the changes in RVEF were only modestly related to the changes in RV load (measured by pulmonary vascular resistance (PVR)). Moreover, despite the fact that PVR is reduced by treatment, RVEF can deteriorate. The decrease in RVEF was associated with poor outcome, independent of the reduction in PVR (Fig. 12.2) [4].


Fig. 12.2
After 1 year of current PAH targeted medical treatment, the changes in pulmonary vascular resistance (PVR) were modestly related to the changes in RVEF (a). In the PAH patients with a reduced PVR after therapy, a decrease in RVEF was associated with a worse survival compared to patients with a stable/improved RVEF. Both groups showed a similar decrease in PVR after treatment (mean PVR reduction: 284 ± 248 dyne s cm5) (b) [4]
The determination of RV ejection fraction is relatively time consuming whereas stroke volume can be more easily obtained by phase contrast mapping MRI [38]. Other more readily applicable measures of RV function have also been explored. RV shortening in a longitudinal plane (tricuspid annular plane excursion or TAPSE) and transverse plane provided simple estimates of RV ejection fraction of which transverse shortening showed the strongest correlation [39]. In addition, Mauritz et al. showed that assessments of both parameters are valuable, but that changes in transverse wall motion rather than changes in TAPSE reflect RV dysfunction during end-stage disease [36].
Regional ventricular wall deformation and ventricular synchrony are important aspects of RV function. MRI tagging techniques are considered as the reference techniques to measure the relative amount of myocardial wall deformation (segmental strain), the velocity of deformation (strain rate), and synchrony (i.e., timing of mechanical activation and relaxation between wall segments); all of these parameters can be determined in a circumferential, longitudinal, and radial axis. It has been found that in the healthy RV, there is a predominant longitudinal rather than circumferential wall deformation, resulting in a bellows-like or peristaltic action. Normal wall deformation is in general larger at the basal and apical segments than at the mid-segment [40]. Patients with PH showed an altered pattern with a globally reduced longitudinal and circumferential wall deformation [41]. Furthermore, it has been found that regional longitudinal wall deformation can already be disturbed at the time that global RV function is still intact, implying that changes in regional measures could be sensitive parameters to detect early RV dysfunction in PAH [42].
In addition, it has been shown by MRI that RV asynchrony may contribute to the development of impaired cardiac function. In PAH patients, RV mechanical contraction is prolonged and continues after the pulmonary valve closes (resulting in a so-called post-systolic contraction period) rendering RV contraction inefficient [43, 44]. In addition, at the time that the RV still contracts, the LV in PAH is already in its relaxation phase, leading to leftward septum bowing, a low LV end-diastolic volume and decreased stroke volume [44–46]. In fact, a low LV end-diastolic volume appears to be a strong predictor of poor prognosis [16].
RV Molecular and Perfusion Imaging
RV Metabolic Remodeling
PET studies have demonstrated that in LV cardiomyopathies a switch takes place from primarily fatty acid metabolism to glucose metabolism in order to maintain the adenosine triphosphate (ATP) supply [47, 48]. In the failing human RV under chronic pressure overload, single photon emission computed tomography (SPECT) has demonstrated a decreased RV uptake of fatty acids which was associated with impaired RV function and poor prognosis [49]. Fatty acid uptake can also be estimated using PET with 11C-palmitate tracers [47, 50] but such studies have yet to be performed in PAH. In addition, RV myocardial glucose metabolism can be measured by PET with 18F-2-deoxy-2-fluoro-D-Glucose (18FDG) tracers [51]. Some studies have demonstrated an increase in the ratio of RV to LV glucose uptake in PAH patients. However it remains unclear whether this ratio is explained by an increased RV glucose uptake [52–54] or a lowered LV uptake [55]. Furthermore, inconsistent results have been reported with respect to the relevance of glucose metabolism in relationship with RV load and function [51, 54–58]. The differences between study results are perhaps due to differences in patient populations, scanning protocols, or data analysis. At this moment, it remains unclear which changes in metabolism occur in the RV of PAH patients and whether these changes can be regarded as adaptive or indicative of pathological remodeling. This area of imaging of RV metabolism will likely be further developed in the coming years.
RV Blood Flow and Oxygen Balance
By use of Using PET with a combination of 15O-labeled tracers (15O-H2O, 15O-CO, and 15O-O2) or by a more practical method using 11C-acetate tracers, RV myocardial oxygen consumption (MVO2) can be estimated [59, 60]. It has been demonstrated that resting MVO2 is significantly elevated in patients with PAH (Fig. 12.2) and this finding is primarily determined by increased pulmonary pressures and an increased heart rate [58, 61]. PAH patients in NYHA class III show a higher MVO2 compared to NYHA class II patients, despite a similar RV power output, which implies that the RV of these patients becomes mechanically inefficient (Figs. 12.3 and 12.4) [58].



Fig. 12.3
A four-chamber (a) and short-axis (b) image obtained by positron emission tomography (PET) with 11C-acetate tracers in the same patient with end-stage RV failure as in Fig. 1. It is demonstrated that part of the RV wall shows an increased myocardial oxygen consumption (MVO2) (bright, yellow) that can be even higher compared to the LV

Fig. 12.4
Impaired RV mechanical efficiency in patients with PAH is primarily determined by increased MVO2. Light gray bars = New York Heart Association (NYHA) Functional class II patients, dark bars = NYHA functional class III patients. Patients in NYHA II showed a higher cardiac output (CO) (a) and similar mean pulmonary artery pressure (mPAP) (b) compared to NYHA III patients. Consequently, RV power output (i.e., product of CO and mean PAP) was similar in both groups (e). RV myocardial blood flow determined by positron emission tomography (PET) with H215O tracers (c) and oxygen (O2) extraction fraction estimated by PET using 15O2 tracers (d) were not statistically different in both groups. However, there was a significantly higher MVO2 per gram of myocardial tissue in NYHA III compared to NYHA II patients (f). RV mechanical efficiency was reduced by ~50 % in NYHA III in comparison with NYHA II as a result of a similar RV power output but higher MVO2 (g). [58] (Wong et al. Circ HF 2011; Reprint Fig. 3)
The normal RV has two mechanisms which generate an increased MVO2 during stress: (1) coronary flow reserve and (2) oxygen extraction fraction (OEF) reserve [62–65]. In patients with PAH during resting conditions, the OEF is already elevated whereas the mean resting myocardial blood flow is similar in PAH compared to controls [58, 66, 67]. In addition, PAH patients show an impaired perfusion reserve since myocardial blood flow did neither increase during adenosine-induced stress MRI [66] nor during PET imaging with cycling exercise [68]. Surprisingly, the change in myocardial blood flow during exercise was unrelated to resting OEF. Especially in patients with a high resting OEF, an attenuated increase in blood flow during exercise was observed and was related to a poorer clinical, hemodynamic and RV condition [66, 68]. The findings of impaired myocardial blood flow reserve might be explained by multiple factors. First, although mean resting coronary blood flow was unchanged, coronary flow was inversely related to the amount of RV hypertrophy [67]. Transmural blood flow may be impaired, perhaps as a consequence of a reduced capillary density of the failing RV tissue [22]. Indeed, impaired angiogenesis might play a role in the mismatch between hypertrophic myocytes and capillaries. Second, the resting coronary blood flow and perfusion reserve are both negatively associated with increased pulmonary pressures [66, 67], which could imply that these extravascular compressive forces may restrict an increase in blood flow. Other explanations could be impaired autoregulatory flow mechanisms [69] and systemic hypotension resulting in reduced coronary driving pressures. However, van Wolferen et al. showed that the latter factor is probably not a major determinant of impaired RV flow in PAH [67].
To summarize, the increased RV oxygen demand in patients with PAH is not adequately compensated for by an increased oxygen supply. As a potential consequence, Gomez et al. observed RV ischemia in PAH patients by SPECT imaging [70].
The Near Future of RV Molecular Imaging
RV Angiogenesis
In PH animal models, impaired angiogenesis in the setting of RV hypertrophy has been demonstrated; might lead to RV ischemia and fibrosis [35, 71]. Vascular endothelial growth factor (VEGF) is a major contributor to angiogenesis and integrins serve as important mediators of angiogenesis. Novel imaging strategies have been developed that can directly measure angiogenesis in vivo using PET with tracers such as 64CU-labeled VEGF121 or 18F arginine–glycine–aspartic acid peptide (RGD) with affinity for the αvβ3 integrins. Angiogenic imaging has successfully been performed in rat models with myocardial infarction [72–74] and in a patient 2 weeks after myocardial infarction [75]. These imaging techniques may become important in the assessment of pathophysiological mechanisms of RV disease processes in vivo in PAH.
RV Apoptosis
Apoptosis is defined as programmed cell death and may play a causal role in the development of cardiac hibernation and heart failure [76]. Radiolabeled annexin can be used as an imaging protein that binds to phosphatidylserine (PS); a phospholipid expressed on the outer cell membrane during apoptosis which serves as a marker for macrophages to remove apoptotic cells [77]. It has been demonstrated by SPECT that increased 99Tc-Labeled annexin V uptake was associated with the deterioration of LV function in patients with LV failure [78]; increased annexin uptake was also related to allograft rejection in cardiac transplant recipients [79]. Such studies have not yet been performed in PAH patients but in PH animal models increased levels of SPECT cardiac annexin V uptake have been detected [80].
In addition, activated caspases are involved in the signal transduction of apoptosis and when radiolabeled, they can be used for PET measurements of apoptosis in vivo. In mouse models of dilated cardiomyopathies, it has been shown that although the overall rate of myocyte apoptosis was low, persistent levels can overwhelm the limited regenerative capacity of the myocardium contributing to heart failure [81].
RV Neurohormonal System
Preclinical studies have demonstrated that dysfunctional neuronal signaling might be an important hallmark in the development of RV failure in PAH [82]. PET imaging with radiolabeled norepinephrine (NE) analogs such as 11C-meta-hydroxyephedrine (HED) can be applied to estimate presynaptic sympathetic function and 11C-CGP-12177 or 11C-CGP-12388 tracers are used to estimate levels of postsynaptic sympathetic β-adrenoceptors. HED reuptake and β-adrenoceptor density were reduced in patients with LV cardiomyopathies and associated with impaired LV function and worse survival [83–85].
The renin–angiotensin–aldosterone system (RAAS) activity in PAH is increased and associated with disease severity [86]. By use of PET imaging with 11C-KR31173 tracers, levels of cardiac angiotensin receptors have been detected in healthy volunteers [87] such data might be of pathophysiological interest also in patients with PAH.
Magnetic Resonance Spectroscopy
Magnetic resonance spectroscopy (MRS) is a technically difficult technique but can provide insight into cardiac metabolism in vivo without requirements of an external tracer. There is limited expertise in the field of PAH [88] but multiple studies have been performed in patients with LV failure. Multiple 31 Phosphorus MRS studies have demonstrated that LV levels of creatine and ATP were decreased in advanced heart failure and related to survival [89].
Hybrid PET-MRI
Currently, hybrid PET-CT has become commonplace in clinical practice and research settings and has significantly contributed to our insights into pathophysiological processes in cardiovascular diseases [90]. Although CT allows detailed structural imaging it is less useful in RV functional assessment. Hybrid PET-MRI is an innovative, rapidly accepted technique which does not only allow (simultaneous) combination of PET with anatomic imaging but also provides functional imaging, perfusion imaging, tissue characterization, and flow imaging that might provide improved insights into the pathophysiology of RV failure. However one limitation of hybrid systems with MRI is that information required for attenuation correction of nuclear images is not provided. Recently, the first hybrid PET-MRI results of patients with myocardial infarction have been published and have demonstrated high image qualities (Fig. 12.5) [91–93].


Fig. 12.5
Short-axis images obtained by hybrid PET-MRI in a patient with myocardial infarction of the septal and anteroseptal wall. MRI with late gadolinium enhancement (LGE) demonstrates the infarct zone with a no-reflow phenomenon (arrows) (a). Fusion of 18F-2-deoxy-2-fluoro-D-Glucose (18FDG) PET and MRI LGE images demonstrates absent 18FDG uptake in the infarcted area (b) [91]. (Nensa et al. Radiology 2013; Reprint Fig. 2)
Summary and Conclusions
We predict that in vivo cardiac MRI and PET will significantly contribute to a better understanding of the pathophysiological processes which lead to the development of chronic RV failure. Imaging studies have demonstrated that in the setting of chronic pressure overload, the RV compensates enduringly to sustain CO by an increase in wall mass, dilatation and contractility, and marked changes in the RV shape. With passage of time, these compensation mechanisms fail, resulting in increased wall stress and impaired global RV function. Other factors that might contribute to disturbed RV function are a reduced wall deformation and an inefficient RV contraction pattern. The resulting interventricular asynchrony is associated with leftward septum bowing, impaired LV filling, and decreased stroke volume. Furthermore, the RV becomes mechanically insufficient: more oxygen is required for a comparable power output. At the same time, RV oxygen delivery is impaired and tissue oxygenation is reduced. Alterations in myocardial metabolism have been observed in PAH, but their overall relevance and cause or consequence of RV failure remains unclear. In the near future it can be expected that the importance of changes in cellular functions and signaling pathways will become clear and “imageable.” This may allow a regional and quantifiable analysis of processes such as angiogenesis, apoptosis, and neurohormonal factors impact. Fig. 12.1 provides an overview of currently available clinical imaging tracers that could be relevant for the assessment of molecular processes of RV diseases in patients. In addition, recent developments in (hybrid) PET and MRI might allow an integrated RV assessment in vivo. They will likely provide an important basis for simultaneous measurements of multiple myocardial disease processes.
Acknowledgements
We would like to thank Dr. Hans Harms for his support of the image collection.
References
1.
Hunt SA, Abraham WT, Chin MH, Feldman AM, Francis GS, Ganiats TG, et al. 2009 Focused update incorporated into the ACC/AHA 2005 guidelines for the diagnosis and management of heart failure in adults a report of the American College of Cardiology Foundation/American Heart Association Task Force on Practice Guidelines developed in collaboration with the International Society for Heart and Lung Transplantation. J Am Coll Cardiol. 2009;53(15):e1–90.PubMedCrossRef
2.
3.
Voelkel NF, Quaife RA, Leinwand LA, Barst RJ, McGoon MD, Meldrum DR, et al. Right ventricular function and failure: report of a National Heart, Lung, and Blood Institute working group on cellular and molecular mechanisms of right heart failure. Circulation. 2006; 114(17):1883–91.PubMedCrossRef
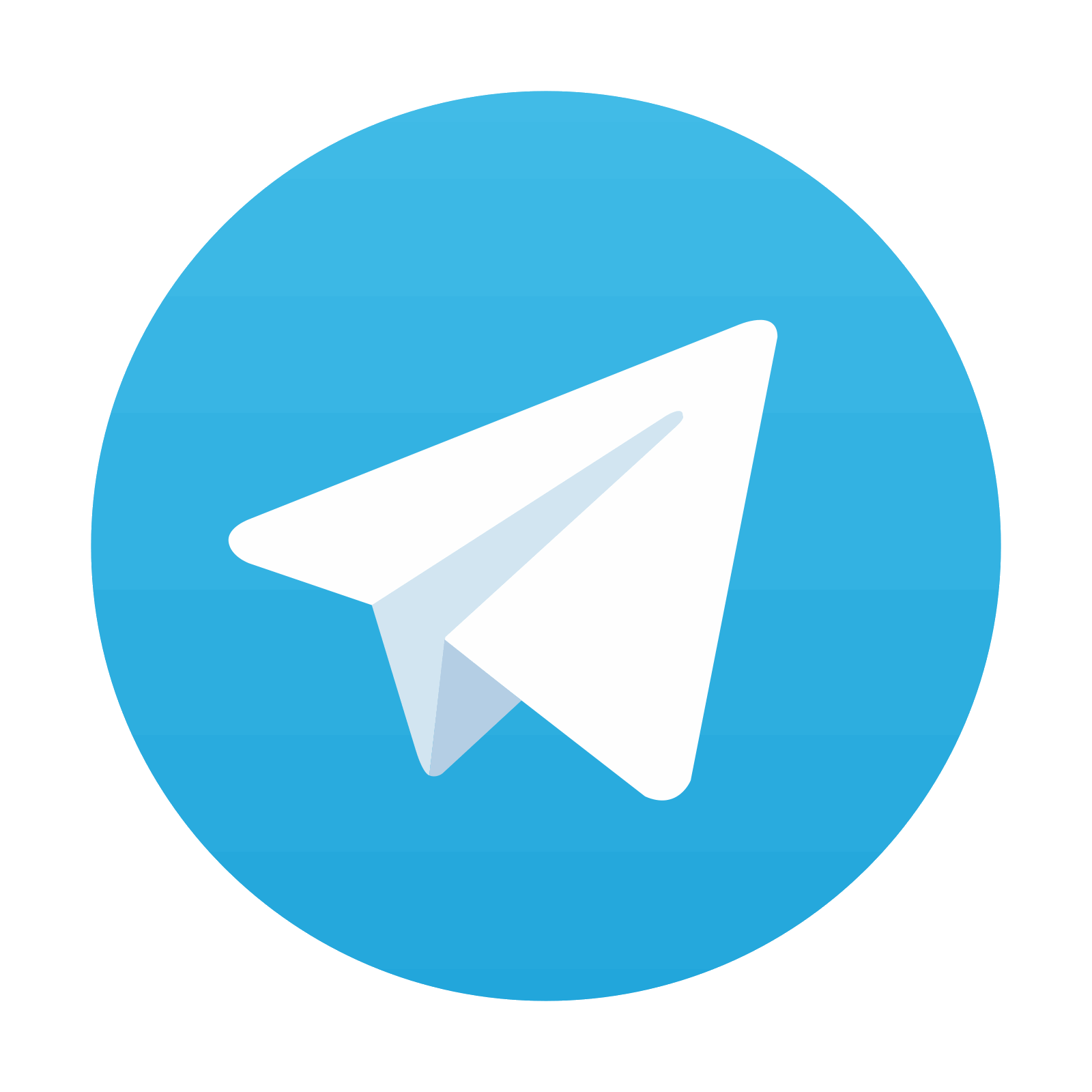
Stay updated, free articles. Join our Telegram channel
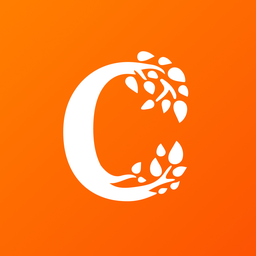
Full access? Get Clinical Tree
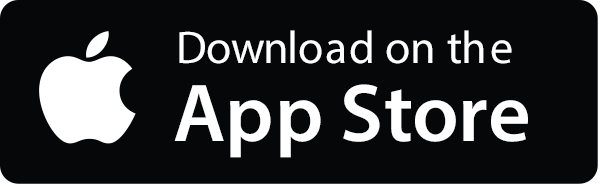
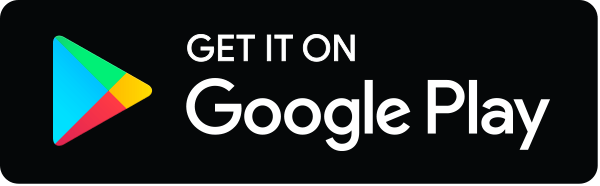