Knowledge of the role of cardiac-specific genes and their modulating factors has increased tremendously over the last decade, although 85% of human congenital cardiovascular disease is still considered to be multifactorial in origin. Advances in the molecular biology of the developing heart have greatly contributed to our understanding of cardiac morphogenesis. Manipulation of conserved genes from a variety of model organisms has increased our understanding of how genetic factors and cellular interactions contribute to cardiac development. Transgenic mouse models have allowed time-specific tracing of cells and their role in heart formation. The problem of embryo-lethality after manipulating “cardiac-specific” genes has been overcome by inducible knockout strategies. Whole genome sequencing programs have also increased understanding of mutations in humans that lead to congenital cardiovascular disease.
This chapter summarizes the initial phases of cardiac development. We then describe in more detail how cardiac morphogenesis leads to formation of the four-chambered heart and how abnormal cardiogenesis contributes to congenital cardiovascular disease.
Heart development starts with two cardiogenic plates derived from the lateral splanchnic mesoderm. These plates fuse in the midline in the anterior (cranial) region of the embryo. The crescent of the cardiogenic plates is referred to as the first heart field (FHF) and is flanked medially by the second heart field (SHF) mesoderm (Figure 1A). Upon fusion in the midline, the FHF forms the two-layered primary heart tube with myocardium on the outside lined by endocardium on the inside. The myocardium secretes a glycoprotein-rich layer, the cardiac jelly, toward the endocardium. The primary heart tube connects to the arterial pole cranially and to the venous pole caudally (Figure 1-1B) but does not contain all segments of the four-chambered heart. Venous tributaries abut on the small atrial component, followed downstream by the future atrioventricular canal and a primitive left ventricle. Finally, the outflow tract connects to the aortic sac at the arterial pole (Figure 1-1C). The various components can be distinguished soon after, as both the AV canal and the outflow tract contain an increasing amount of cardiac jelly that forms the endocardial cushions. The cushions become even more prominent as they acquire mesenchymal cells, derived from the endocardial lining as a result of endocardial-mesenchymal transition. Subsequently, the primary heart tube starts the developmentally determined rightward looping.
FIGURE 1-1.
Cardiac development. A. Schematic depiction of the precardiac mesoderm in the primitive plate. The brown area reflects the mesoderm of the FHF, whereas the yellow area corresponds to the putative SHF mesoderm. B. Primary heart tube derived of FHF mesoderm. The tube consists of myocardium, lined by cardiac jelly. C. Heart tube after looping. The yellow areas reflect SHF-derived contributions. The SHF contributions to the outflow tract have not been depicted. Note that in this stage the atria are still positioned entirely above the primitive left ventricle, whereas the outflow tract is positioned above the primitive right ventricle. D. Advanced stage of heart development. Septation has now occurred at the level of the atria, ventricles, and outflow tract. E. Sagittal view of an embryo. The (anterior and posterior) SHF mesoderm and its derivatives are depicted in yellow. Contributions from neural crest cells are depicted in blue. A proepicardial organ can be distinguished at the venous pole as well as a smaller proepicardial organ at the arterial pole. F. Scanning electronic microscopic section at the level of the venous pole in a chick embryo, showing the venous pole of the proepicardial organ. G. Scanning electronic microscopic section at the level of the outflow tract in a chick embryo, showing the arterial pole of the proepicardial organ (arrowheads, asterisk). Abbreviations: A, atrium; Ao, aorta; AoS, aortic sac; aPEO, atrial pole of proepicardial organ; AVC, atrioventricular canal; EC, endocardial cushions; FHF, first heart field; HH, Hamburger and Hamilton; IVC, inferior vena cava; LA, left atrium; L, liver; LCV, left cardinal vein; LV, left ventricle; LVV, left venous valve; PAA, pharyngeal arch artery; PC, pericardial cavity; PT, pulmonary trunk; PV, pulmonary vein; RA, right atrium; RCV, right cardinal vein; RV, right ventricle; RVV, right venous valve; SHF, second heart field; SV, sinus venosus; SVC, superior vena cava; VPEO, venous pole of proepicardial organ. B–E: Adapted from: Gittenberger-de Groot AC et al. Ann Med. 2014;46(8):640-652. F and G: Adapted from: Gittenberger-de Groot AC et al. Differentiation. 2012;84(1):41-53.

At the same time, the SHF adds progenitor cells to both the venous and the arterial poles, which ultimately form the essential components of the right ventricle (RV) and at least a part of the right side of the interventricular septum (Figure 1-1D). At the venous pole, the SHF forms cardiomyocytes encapsulating the sinus venosus and its tributaries. The sinus venosus is incorporated subsequently into the wall of the right and left atrium. Likewise, the walls of the great arteries, the embryonic pharyngeal arch arteries that connect to the aortic sac, are partly built from SHF-originating cells. Neural crest cells also contribute to formation of the great arteries, as will be explained below in this chapter.
Multiple specific transcription factors and signaling molecules are essential to the early stages of cardiogenesis. These include the earliest markers of the precardiac mesoderm, including the homeobox-containing gene Nkx2.5 and the zinc-finger-containing GATA4/5/6 subfamily. Members of the T-box family, TBx1/5/18/20, also have essential roles in SHF differentiation. Myocardial differentiation is regulated by several myocyte-specific genes, including myosin light and heavy chain, alpha-cardiac actin, and cardiac troponin I.
The neural crest cells are an ecto-mesodermal derivative arising from the crest of the neural tube, migrating toward various parts of the embryo, including the heart. Cardiac neural crest cells differentiate into cells of the autonomic nervous system and into vascular smooth muscle cells of the pharyngeal arch arteries and contribute to the arterial pole of the heart entering the endocardial outflow tract cushions (Figure 1-1E). Neural crest cells within the heart are involved in modulation and induction of semilunar valve formation and generation of the myocardial component of the outflow tract septum. At the venous pole, where their contribution is less important, a similar effect is observed in the atrioventricular cushions. More recently, cardiac neural crest cells contributing smooth muscle cells to the coronary arteries have been identified.
The epicardium (splanchnic mesodermal lining of the pericardial cavity) is a secondary layer covering the myocardial tube and the intrapericardial part of the arterial pole. During embryonic development, the epicardium originates from both the venous and the arterial poles (Figure 1-1E–G). The larger epicardial population derives from a protrusion of the coelomic wall, covering the sinus venosus and liver primordium (Figure 1-1F). The cells of the proepicardium spread along the outer wall of the ventricles and atria to the border of the myocardium at the arterial pole. Here, they join the arterial epicardium, which is derived from a much smaller arterial proepicardium exhibiting a slightly different phenotype (Figure 1-1G). On activation, the epithelial epicardium undergoes endocardial-mesenchymal transition, and the resulting mesenchymal cells fill the subepicardial space as epicardium-derived cells. These cells migrate between the myocardial cells of the heart tube, the atrioventricular cushions, and the future fibrous annulus. These epicardium-derived cells, in contrast to neural crest cells, differentiate into several cell lines including the majority of the cardiac fibroblasts and the vascular smooth muscle cells of the coronary vascular system (Figure 1-2). The endothelial lining of the coronary vascular system is derived from the endothelium of the sinus venosus/liver primordium adjacent to the proepicardium (Figures 1-1F, 1-2).
FIGURE 1-2.
Cell lines contributing to the developing heart. Schematic representation of cardiac cell lines (cardiomyocytes, endocardium, epicardium, and endothelium) that are derived from the first and second heart field mesoderm and are the main contributors to the definitive heart and vessels. Epicardium-derived cells (in yellow) as well as inadequate interaction with other cardiac cell types may play a role in some cardiac malformations (green boxes). The second heart field and neural crest cell contribution to the great vessels is not represented. Abbreviations: CMPCs, cardiomyocyte progenitor cells; EPDCs, epicardium-derived cells; ECs, endothelial cells; VSMCs, vascular smooth muscle cells. Adapted from: Gittenberger-de Groot AC et al. Differentiation. 2012;84(1):41-53.

Starting with the developmentally determined rightward looping, it is clear that the heart and its connections to the lungs are not symmetric. Situs inversus and heterotaxy occur in humans; several mouse models have increased our knowledge on essential signaling factors related to determination of situs. It is remarkable that only the atrial situs and its contributing posterior SHF seem to be influenced by these factors, while right ventricle and left ventricle with their specific morphologies do not copy the atrial situs anomalies (eg, inversus or ambiguous).
In the preceding paragraphs, the cellular building blocks of the cardiovascular system have been presented. Serious disturbances of one or more cell populations can lead to abnormal cardiac development and even early embryo-lethality. This results in early spontaneous abortion in humans. In homozygous mouse strains, 50% of spontaneous early embryo-lethality related to mutations is caused by cardiovascular abnormalities. In the usually nonhomozygous human, the percent of spontaneous abortions caused by congenital cardiovascular disease cannot be determined unequivocally. The FHF lesions are considered to be the most critical for embryonic demise. Most of the cardiac malformations that clinicians encounter in the perinatal period occur during looping and events mediated by the SHF. Spontaneous abortion in the second trimester caused by congenital cardiovascular disease is less common, as most forms of cardiac malformations are compatible with intrauterine survival.
In the human embryo, the formation of the four-chambered heart occurs by about 8 weeks’ gestation. Thereafter, maturation and remodeling of, eg, the pharyngeal arch arteries and valves are essential for ensuing proper functioning and postnatal survival. The most important elements of cardiac morphogenesis (summarized in Figure 1-3), including septation, valve formation, conduction system maturation, and coronary vascular development, will be presented.
FIGURE 1-3.
Cardiac morphogenesis. Schematic representation focusing on the time line and the major events during cardiac morphogenesis. Many processes essential to heart formation overlap during the 7 to 8 weeks of development, making it difficult to determine the separate molecular pathways or the primary insult that leads to congenital cardiovascular malformations, either isolated or complex. Valvulogenesis occurs relatively late in heart formation, while completion of atrial septation (closure of the foramen ovale) and ductus arteriosus differentiation (closure of the ductus arteriosus) naturally occur after birth because of the unique requirements of the fetal circulation. Source: Jongbloed MRM, et al. Development of the Cardiac Conduction System and the Possible Relation to Predilection Sites of Arrhythmogenesis, TheScientificWorldJOURNAL, vol. 8, pp. 239-269, 2008.

The precardiac mesoderm of the SHF at the venous pole develops uniquely from an Nkx2.5-negative but myosin light chain positive cell population, surrounding the lumen of the sinus venosus. This myocardial cell lineage is incorporated into the posterior wall of both the right and the left atria. The atrial appendages are probably related to the FHF.
The left and right cardinal veins (the embryonic superior and inferior caval veins) are incorporated into the right atrium and are flanked by the folds of the embryonic right and left venous valves (Figure 1-1C, D). The left inferior cardinal vein (future coronary sinus) and the left superior cardinal vein (regressing in the human heart as the ligament of Marshall) all drain into the cavity of the right atrium. A splanchnic vascular plexus surrounds the developing lung buds. During early developmental stages, the primary route of pulmonary drainage from this plexus is toward the systemic veins; a direct connection of the primitive pulmonary veins to the heart is achieved from different tissue later during development. The anlage of the primitive pulmonary vein, the so-called mid-pharyngeal endothelial strand, initially does not have a lumen and is connected to the sinus venosus. During atrial septation it connects to the dorsal wall of the left atrium. The splanchnic pulmonary venous connections with the systemic cardinal (putative caval) veins gradually disappear during normal development.
During atrial septation, four components deserve attention: the primary septum, the dorsal mesenchymal protrusion, the septum secundum, and the endocardial cushions. The initially two-layered myocardial primary atrial septum is positioned between the right and left atria. It partially disintegrates forming the ostium secundum, which is required for the formation of the embryonic foramen ovale (Figure 1-4A, B), the essential communication between the right and left atria during fetal life. Fusion of a mesenchymal cap on the free rim of the primary atrial septum with the atrioventricular endocardial cushion mass and with the dorsal mesenchymal protrusion closes the ostium primum. This structure is found at the right side of the primary atrial septum as a thick mesenchymal mass (Figure 1-4A, B) and will develop into the muscular base of the atrial septum. At the right side of the primary septum and usually incorporating the left venous valve, a folding process of the atrial myocardial wall forms the crescent ridge of the atrial septum secundum (the limbus). During development, the free edge of the primary atrial septum (the valve of the foramen ovale) and the rim of the septum secundum (the limbus) will overlap, allowing blood to pass via the foramen ovale (Figure 1-4B). At a variable time after birth, these two rims often fuse, resulting in the closure of the foramen ovale, although this structure remains patent in up to 20% of normal adults.
FIGURE 1-4.
Atrial septation. A. Early development of the dorsal and cranial wall of the right and left atria. The gold indicates the contribution of the SHF to the incorporated sinus venosus myocardium. The appendages are not depicted. Both the inferior and the superior vena caval veins enter in the right atrium as well as the coronary sinus, which is derived from the left superior cardinal vein. A mesenchymal cap (green) under the rim of the primary atrial septum borders the ostium primum, which connects the right and left atria. The entrance of the primitive pulmonary vein is seen in the left atrium. The infolding of the superior wall of the right atrium that will form the secundum atrial septum is already seen merging with the dorsal mesenchymal protrusion. B. After completion of septation, the ostium primum is closed by fusion of the mesenchymal cap with the atrioventricular cushions, which have now divided the atrioventricular canal into a tricuspid and a mitral orifice. The perforations in the primary atrial septum have enlarged to form an ostium secundum that in combination with the free rim of the secundum atrial septum is part of the foramen ovale (arrow) that closes after birth. Abbreviations: AVC, atrioventricular canal; DM, dorsal mesocardium; IVC, inferior vena cava; LA, left atrium; MC, mesenchymal cap; MO, mitral orifice; OP, ostium primum; OS, ostium secundum; PAS, primary atrial septum; PV, pulmonary vein; RA, right atrium; SAS, secundum atrial septum; SVC, superior vena cava; TO, tricuspid orifice. Used with permission from Gittenberger-de Groot AC, et al., (2011). Normal and Abnormal Cardiac Development. In: Pediatric Cardiovascular Medicine, Second Edition (eds JH Moller and JIE Hoffman), Wiley-Blackwell, Oxford, UK.

As described above, pulmonary drainage is initially via an extensive midsagittal splanchnic vascular network. Disturbance of the SHF can lead to misalignment and faulty incorporation of the primitive pulmonary veins into the dorsal left atrial wall. In case of absence or atresia of the mid-pharyngeal endothelial strand, either the early pulmonary to systemic connections will persist or abnormal connections will develop, leading to abnormal drainage of the pulmonary venous blood to three levels: subdiaphragmatic (eg, scimitar syndrome; Figure 1-5A), cardiac, and supracardiac (Figure 1-5B). The drainage of all the pulmonary veins can be abnormal (total anomalous pulmonary venous connection) or partial, with some pulmonary veins entering in into left atrium and some into either systemic veins (Figure 1-5B) or the right atrium. The few known genetic causes are linked to abnormalities of left/right asymmetry (heterotaxy) caused by mutations of the transcription factor PITx2 or by more downstream signaling abnormalities (eg, PDGFRα).
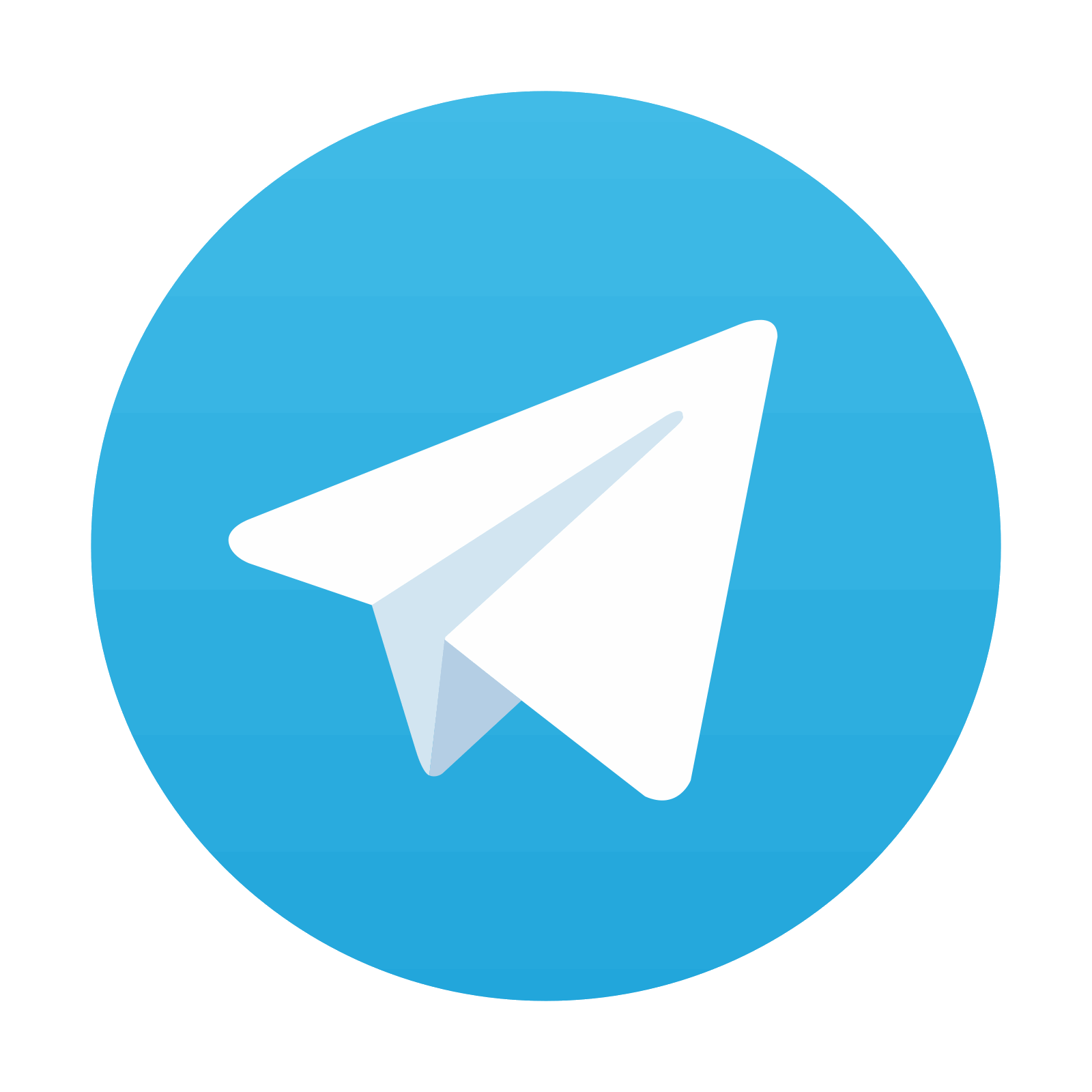
Stay updated, free articles. Join our Telegram channel
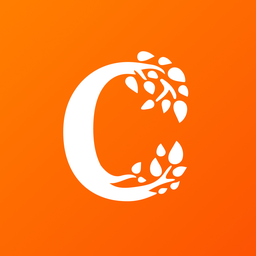
Full access? Get Clinical Tree
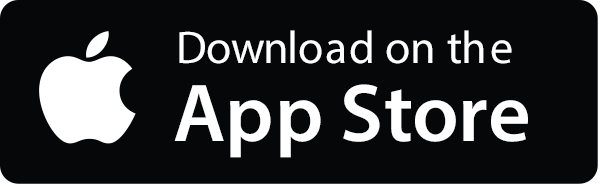
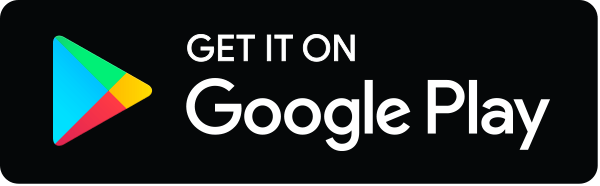