Chapter 1
Cardiac investigations
Exercise ECG
A commonly used test involving a treadmill, blood pressure (BP) measurement, and continuous electrocardiograph (ECG) monitoring. Overall sensitivity for coronary heart disease is around 68% and specificity is 77%. This increases when considering prognostically significant disease, which has a sensitivity of 86%. The test improves to have a predictive accuracy of >90% in intermediate- to high-risk patients (older men with ischaemic symptoms). The test is of least value in populations that are least likely to be suffering from ischaemic heart disease; e.g. asymptomatic middle-aged women have a positive predictive value of <50%.
Indications
Contraindications
When to stop
Criteria for a positive test
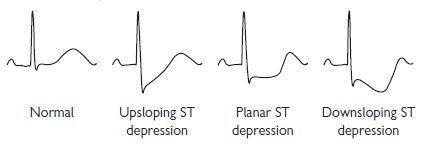
*Features that are indications for urgent angiography: Also ST depression at low workload (<<6 minutes Bruce) in multiple lead groups, persisting into recovery, >2 mm, downsloping pattern.
Causes of false-positive tests
National Institute for Health and Clinical Excellence (NICE) guidance 2010—chest pain of recent onset1
Historically, exercise ECG testing has been the cornerstone of investigation of patients presenting with stable anginal chest pain. However, sensitivity and specificity are poor compared with other investigations. Recent UK guidelines discouraged the use of exercise ECG in patients without known coronary artery disease.
Instead, NICE recommended cardiac computed tomography (CT) for patients with low likelihood of significant coronary artery disease, a functional test such as dobutamine or exercise echocardiography for those with intermediate likelihood, and invasive coronary angiography for those with high likelihood.
Cardiac computed tomography
In the past decade, major advances in CT technology have enabled cardiac CT to emerge as a non-invasive alternative to conventional invasive coronary angiography.
Coronary artery calcium scoring
Calcified coronary plaque represents approximately 20% of the total coronary artery plaque burden. Therefore, the degree of coronary calcification can be used as a surrogate for coronary artery atherosclerosis. A calcium score is obtained through a low-radiation unenhanced (i.e. no iodinated contrast) scan. The degree of calcification in each coronary vessel is then expressed as a figure and summed to give the total coronary artery calcium score, commonly using the Agatston scale. A score of 0 correlates with a low risk of coronary artery disease, whereas a score of >400 correlates with high risk of significant atherosclerotic plaque burden.
Clinical studies have shown that the calcium score provides additional information for cardiovascular risk stratification above and beyond traditional factors such as age, sex, hypertension, family history, hyperlipidaemia, and diabetes.
CT coronary angiography
CT coronary angiography is performed by injecting iodinated contrast into the peripheral veins. When the level of contrast in the aorta reaches a specified level, the scan is launched and images are acquired. The images are then analysed using computer software, which allows manipulation in two dimensions and three-dimensional (3D) reconstruction of the coronary arteries and cardiac chambers, also known as ‘volume rendering’.
Technical considerations
Temporal versus spatial resolution
The principal challenge to imaging the coronary arteries using CT is to achieve high temporal resolution. Temporal resolution is defined as the time taken to acquire an image. As the beating heart moves, it needs to be ‘frozen’ during image acquisition. Motion is greatest during systole, whereas the heart is relatively still during diastole. Images acquired during diastole are therefore most likely to be motion free, allowing accurate interpretation and diagnosis. A CT scanner must therefore be capable of acquiring images rapidly during the short diastolic phase when the heart is motionless.
Imaging small structures such as coronary arteries requires high spatial resolution. Spatial resolution is defined as the narrowest distance between two objects that can be distinguished by the detector. CT spatial resolution is determined by the voxel size (i.e. 3D pixel).
Heart-rate control
The duration of systole is relative fixed at different heart rates, but the duration of diastole varies greatly. A slow heart rate (i.e. <65 bpm (beats per minute)) is preferable, to ensure motion-free imaging of the coronary arteries.
Strategies for reducing radiation effective dose
A number of strategies have emerged to reduce the effective dose of ionizing radiation per scan:
By combining these techniques, the effective dose for CT coronary angiography is now approaching 1 mSv (milli-Sievert).
Sensitivity and specificity
Modern 64-slice and above CT scanners have been shown to provide excellent sensitivity (95%) and specificity (83%). As a consequence, CT coronary angiography has a very high negative predictive value (upwards of 95%). The principal clinical application of CT coronary angiography is to rule out significant coronary artery disease in patients with low to intermediate cardiovascular risk.
Clinical applications of cardiac CT
Comparison with traditional invasive coronary angiography
Traditional cardiac catheterization remains the gold standard for diagnosing coronary artery disease. It has excellent temporal and spatial resolution and allows for intervention if significant disease is identified. However, it is invasive and exposes the patient to potential vascular complications, including MI, stroke, and vascular-access complications.
With new 256- or 320-detector CT, the extended coverage and scan time of less than 0.5 s allows the entire heart to be imaged in a single heartbeat. Compared with conventional angiography, CT has a lower spatial resolution (0.4–0.6 mm vs. 0.2 mm) and temporal resolution (60–220 ms vs. 8 ms).
Comparison with functional tests
Historically, non-invasive functional tests (e.g. exercise tolerance testing, stress echocardiography, nuclear imaging, positron emission tomography (PET), perfusion magnetic resonance imaging (MRI)) have been used to select those patients deemed at intermediate risk who are likely to require invasive coronary angiography and intervention. However, many of these tests are labour intensive and not all are readily available in every hospital. In addition, the false-positive rate is substantial, leading to patients with normal coronary arteries undergoing unnecessary cardiac catheterizations.
Indications for cardiac CT
The principal role of cardiac CT in current clinical practice is to rule out or detect coronary artery disease. CT is particularly good at assessing anomalous coronary arteries and coronary artery bypass graft patency.
Additional indications
These include:
Advantages and disadvantages of cardiac CT
Advantages
Disadvantages
Future applications of cardiac CT
CT perfusion imaging
New CT techniques are now enabling integration of anatomical assessment of the coronary arteries with functional information. Intravenous contrast is injected and the myocardium is scanned repeatedly over a period of time. The first pass of contrast through a region of interest is tracked to produce tissue-specific time–density curves, which are then interpreted to determine blood flow within the tissue. However, using current CT technology, the predominant barrier to use of CT perfusion in routine clinical practice is high radiation doses.
Multimodality hybrid imaging
An alternative to CT perfusion imaging is integration of the anatomical information from CT angiography with the functional information from stress echocardiography, single photon emission computed tomography (SPECT), PET, or perfusion MRI. Clinical studies have shown that fusing functional and anatomical information increases the sensitivity and specificity when compared with each modality in isolation.
Transthoracic echocardiography
Introduction
Despite dramatic advances in new cardiac imaging technologies, echocardiography remains the most important diagnostic imaging tool in clinical practice. Since its development by Edler and Herz almost five decades ago, and routine clinical implementation a decade later, echocardiography has developed into an intuitive, comprehensible, and practical method to rapidly and repeatedly evaluate cardiac morphology and function. Competent interpretation of the echocardiographic examination first requires an understanding of the physical principles underlying the various technique modalities.
Ultrasound physics
All forms of ultrasonic imaging are based on generation of high-frequency (>1 MHz) acoustic pressure waves from a transducer comprising one or more piezoelectric crystals. Current is passed across the latter, leading to material deformation and wave transmission. The piezoelectric element also serves as a receiver, and waves returning from insonified objects (e.g. walls, valves) deform the crystal(s), which, in turn, generate a current that can be sampled over time. Because the velocity of sound is constant, object location (spatial resolution) can be determined based on the timing of the returning signal. The amplitude of the returning signal is based on the angle of incidence (surfaces perpendicular to the ultrasound beam are stronger reflectors) and the interface of acoustic impedances (greater differences such as occur in the left ventricle at the tissue–blood interface lead to greater reflectivity). Returning ultrasound information is processed for maximum image integrity and then mapped to pixels for display and storage. While many institutions continue to store images on videotape, image degradation necessarily incurred by this medium, as well as ease-of-use issues, have led to increased implementation of digital storage, primarily on dedicated file servers.
M-mode
This was the first available form of echocardiography and, while still available on modern machines, has largely disappeared from routine use in modern laboratories. M- or ‘motion’-mode images depict a single line of ultrasound over time (Fig. 1.1). The information is graphic in nature and requires considerable experience for accurate interpretation. Its advantage lies in its high sampling rate (>1 kHz) and resultant ability to depict rapidly moving structures that may be of interest from a didactic or physiological perspective.
Two-dimensional (2D) or sector scanning (Fig. 1.2)
When an ultrasound beam is swept across a chosen cardiac window, rapid sequential sampling can be performed, leading to display of multiple ‘scan lines’ of information and a sector image created nearly instantaneously. Since a finite number of scan lines is possible, interpolation of data between lines is performed and an image slice or sector (hence the term ‘sector scanning’) is stored in digital form. Through reiterative acquisition over a cardiac cycle, a movie composed of sequentially acquired sectors is created, demonstrating structural motion, which can then be displayed on a monitor. Beam sweeping can be performed by mechanical rotation of one or more crystals, or through the use of programmed firing of a bank of crystals (phased array). Sampling rates were previously dictated and limited by videotape standards (e.g. PAL (phase alternating lines) or NTSC (National Television Standard Committee)) but, with digital capabilities appearing in some form on virtually all machines and replacing outdated tape technology, higher frame rates are possible.
The availability of harmonic tissue imaging has substantially improved image resolution by eliminating artefactual ‘noise’. Low-level signals emanating from tissue and comprising the first harmonic of the transmitted ultrasound are selectively sampled. In this way, extraneous reflections such as reverberations are filtered out, leaving a cleaner image.
Three-dimensional (3D) imaging (Fig. 1.3)
While two-dimensional (2D) ECHO presents user-selected sector or tomographic information, 3D ECHO has the potential to provide a comprehensive evaluation of cardiac anatomy similar to that of more quantitatively mature technologies such as MRI or CT. Three-dimensional ECHO can be performed using the ‘freehand’ approach, in which multiple 2D sectors are acquired from a probe that is positionally mapped using a ‘spark gap’ or magnetic tracking system. Both image and position data are stored for post-hoc 3D rendering. The development of rotational and, more recently, matrix array probes, coupled to powerful computer technology, has made post-hoc, 3D chamber reconstruction a reality, with accurate volumetric assessment. Most recently, real-time rendering of spatially limited cardiac segments has been made available. There are considerable limitations, and it is currently available in limited form from various manufacturers.
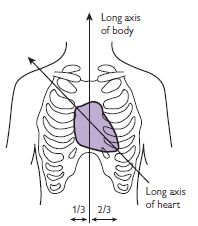
Fig. 1.3 The heart lies in the mediastinum with its own long axis tilted relative to the long axis of the body. Appreciation of this discrepancy is important in the setting of cross-sectional echocardiography. Reprinted with permission from Anderson RH, Ho SY, Brecker SJ (2001). Anatomic basis of cross-sectional echocardiography. Heart 85: 716–20.
Transthoracic Doppler imaging
Quantification of object motion within the heart is performed using Doppler-based technologies. In brief, the same equipment described earlier propagates ultrasound, which is aimed at moving red blood cells or tissue.
Doppler is restricted in its ability to sample high velocities by the Nyquist limit, which is dependent on the sampling rate (the lower the frequency, the higher the evaluable velocity) and object depth (the deeper the object, the lower the sampling rate). When the frequency shift of moving objects (i.e. velocity) exceeds the Nyquist limit, aliasing occurs, precluding velocity assessment.
Pulsed Doppler permits accurate sampling of blood velocities averaged within a limited region of interest or ‘sample volume’. Transducer elements serve as both transmitters and receivers, permitting selective sampling of reflected ultrasound and accurate range or spatial information. Pulsed Doppler spectral displays portray velocity vectors over time:
Flow quantitation from pulsed Doppler
Quantitation: pulsed Doppler spectral traces can provide important information regarding flow quantitation or timing, e.g. assuming that sampling occurs at an orifice with a relatively fixed area over the cardiac cycle (e.g. the left ventricular outflow tract) by integrating the time–velocity spectral curve (‘time–velocity integral’ or TVI), the area under the curve can be multiplied by the area of the orifice (determined from 2D ECHO) and stroke volume determined.
Imaging CW Doppler permits beam steering. Transducer elements are shared for the purposes of CW and intermittent 2D imaging. A virtual cursor positioned over the 2D image can be moved to guide and minimize off-axis sampling angulation. CW Doppler velocities are depicted as a filled-in spectral tracing, since these represent sampling of all of the velocities in structures along the sampling cursor (or ultrasound beam). Blood cells travelling at the fastest velocities are represented at the outer edge of the spectral trace (peak) or darkest line of the trace (modal) velocities.
Colour Doppler flow imaging employs multigate pulsed Doppler to portray blood flow overlying the 2D image. Information is used to detect regurgitant or stenotic lesions or shunts, and qualitative assessment of velocities is possible using colour maps.
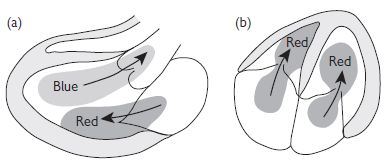
Fig. 1.4 Colour Doppler (Red: toward flow; Blue: away flow). Reproduced with permission from Shanewise JS. Journal of the American Society Echocardiography 1999;12:884–900.
Tissue Doppler imaging (TDI) is used to assess low-velocity displacement of structures. A high-pass filter excludes higher-frequency shifts caused by red-cell flow, leaving only low-velocity shifts attributable to wall motion.
Strain rate imaging can measure regional thickening and thinning, independent of the external influences described above, which influence tissue Doppler measurements. With strain rate imaging, two sampling sites are simultaneously acquired and inter-sample displacement (strain) over time (strain rate) can be determined.
Calculations from Doppler measurements
Valve gradients. The velocity of blood cells travelling across a narrow orifice is directly proportional to the pressure gradient at that point in time. This relationship is approximated by the simplified Bernoulli formula:

In situations where there are high flow velocities (e.g. aortic stenosis with high LVOT velocities), the flow before the stenosis must be accounted for.

Both peak and mean gradients can be determined, the latter by integrating the velocity spectra over a cardiac cycle.
Valve area (continuity equation). This is based upon the principle that flow in the pre-valve area (e.g. LVOT) = flow across the valve. It is generally used for aortic stenosis quantitation, though it is applicable to other stenotic orifices as well.
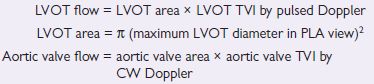
As aortic valve flow = LVOT flow,

PLA = parasternal long axis.
The standard transthoracic ECHO
Most laboratories employ imaging protocols incorporating acquisition of images from standard windows, resulting in standard views presented in a fairly standard sequence. Image quality is maximized by avoiding pulmonary artefacts and rib reflections. Pulsed and colour flow Doppler are performed following 2D imaging in each view. Standard ECHO windows and views include:
Left parasternal window
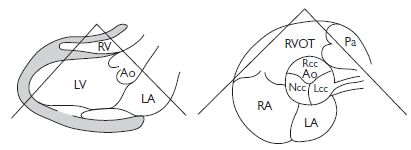
Fig. 1.5 (a) Parasternal long axis view; (b) Parasternal short axis view (aortic level). Reproduced with permission from Shanewise JS. Journal of the American Society Echocardiography 1999;12:884–900.
Apical window
Chamber evaluation
The standard transthoracic ECHO: continued
Left atrium
Left ventricle
Aortic root and ascending aorta
Linear measurements from 2D PLA view or M-mode. The root is measured at cuspal level, and the aorta is measured at the sinotubular junction and 2 cm above this.
Right atrium
Right ventricle
Pulmonary artery
PTP medial–lateral measurement in PSA at aortic valve level.
Assessment of wall motion
Assessment is based upon review of multiple cuts as described earlier. In general, abnormalities of LV regional function should be apparent in more than one view. Various labelling systems have been used but the American Heart Association (AHA) Cardiac Imaging Committee 17-segment model is the standard convention:
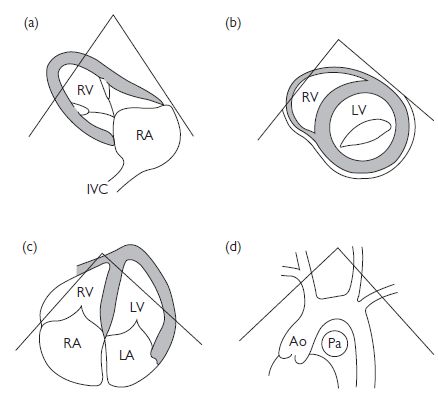
Fig. 1.6 (a) Parasternal long-axis view (RV inflow); (b) parasternal short-axis (mitral valve level); (c) apical 4-chamber view; (d) suprasternal long axis. Ao = aorta; IVC = inferior vena cava; Pa = pulmonary artery. Reproduced with permission from Shanewise JS. Journal of the American Society Echocardiography 1999;12:884–900.
Regional functional assessment
Assessment of LV systolic function
Global functional assessment
Table 1.1 Assessment of left ventricular ejection fraction
LV ejection fraction | Qualitative assessment |
>75% | Hyperdynamic |
55–75% | Normal |
40–54% | Mildy reduced |
30–39% | Moderately reduced |
<30% | Severly reduced |
Essential aspects of ECHO assessment in patients with impaired LV systolic function
Anatomy
Doppler characteristics
Implications for therapy
Assessment of LV diastolic function
Evaluation of LV diastolic function includes measurement of the various phases of diastole including isovolumic relaxation, early filling, diastasis (atrioventricular pressure equilibration without LV filling), and atrial systole. These measures are generally age and heart-rate dependent, and affected by LV loading conditions to variable degrees (see Fig. 1.8).
Pulsed Doppler mitral inflow: E wave (peak early diastolic velocity), A wave (peak atrial systolic velocity), E/A ratio and transmitral deceleration time. The following should be taken into account when assessing these parameters:
Pulsed Doppler of pulmonary veins: Flow into the left atrium can be sampled. S, D, and A waves represent systolic and diastolic atrial filling. The A wave is generated by reverse flow into the pulmonary veins during atrial contraction.
CW Doppler of LV outflow/mitral inflow: Isovolumic relaxation time.
Tissue Doppler of medial and lateral mitral annuli (E’, A’)
Colour Doppler flow propagation (CFP) slope: Rate of blood acceleration into the LV from the annulus to the apex.
Various diastolic profiles combining some of the above parameters have been suggested as indicators of degree or stage of ‘diastolic dysfunction’. (see Fig. 1.8). In general:
Echocardiography in aortic stenosis
Aetiology
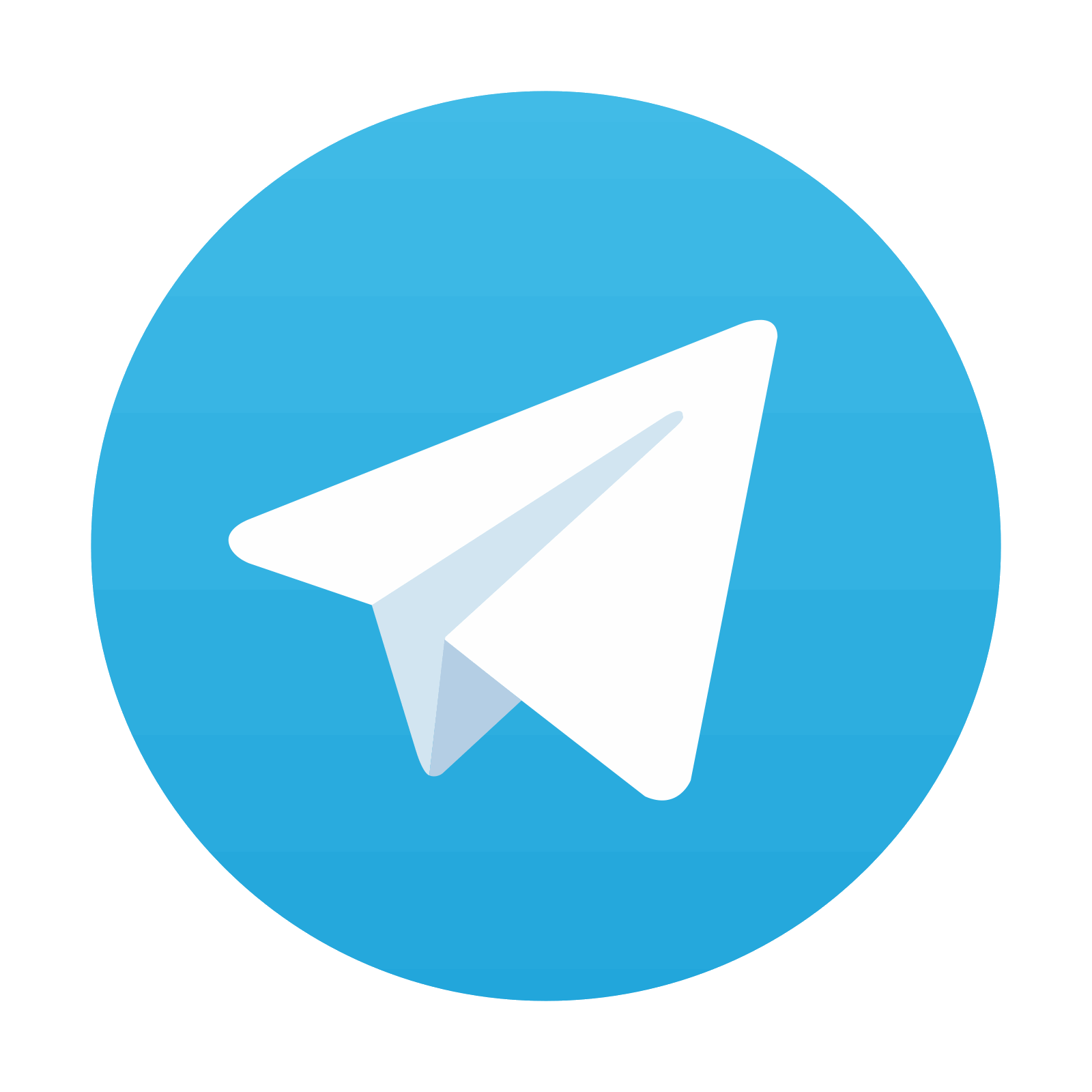
Stay updated, free articles. Join our Telegram channel
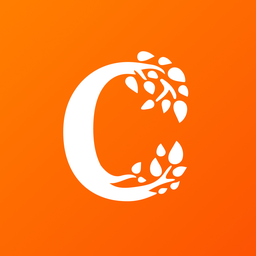
Full access? Get Clinical Tree
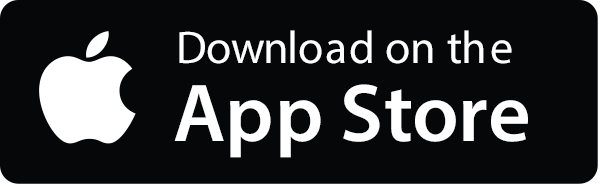
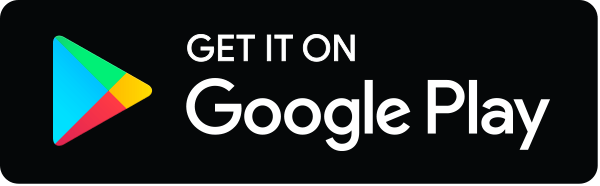