Abstract
This chapter discusses the roles of both diagnostic and therapeutic cardiac catheterization in the management of critically ill infants and children. Providers caring for these patients should be comfortable interpreting invasively acquired hemodynamic data and should recognize the limitations of catheterization data and associated flow and resistance calculations. It is also important to understand indications for both diagnostic and therapeutic cardiac catheterization and to be able to carefully consider the risk-benefit equation for any given patient for whom cardiac catheterization may be considered.
Key Words
Therapeutic cardiac catheterization, hemodynamics, indications, cardiac output, pulmonary vascular resistance, cardiac shunts, angiography
Diagnostic Cardiac Catheterization
The role of congenital cardiac catheterization has evolved since its introduction in the 1940s. Advances in noninvasive imaging have decreased the role of catheterization as a primary diagnostic modality, yet the number of and indications for cardiac catheterization have increased substantially. Partly this is attributable to a burgeoning number of transcatheter interventions that have markedly expanded the role of therapeutic cardiac catheterization. However, diagnostic cardiac catheterization remains an important tool in the assessment of many different cardiac lesions and conditions. In contemporary reports from multicenter registries, approximately half of all congenital cardiac catheterizations are diagnostic procedures without any intervention. This underscores the importance of thorough hemodynamic evaluation and accurate interpretation of data. However, it is also important to understand the limitations of cardiac catheterization, which provides only a snapshot assessment of physiology with hemodynamic data typically obtained under nonphysiologic conditions (e.g., general anesthesia, mechanical ventilation). Moreover, flow, shunt, and resistance calculations have several potential sources of error. For these reasons, data obtained from a cardiac catheterization should always be interpreted cautiously and considered in the context of the patient’s clinical course.
Assessment of Saturation Data
Saturation data are used to calculate flows and to estimate intracardiac shunting. Only four principal saturation data points are needed—a systemic and mixed venous saturation to calculate systemic flows, and a pulmonary arterial and pulmonary venous saturation to calculate pulmonary flows. In a patient with no intracardiac shunting the pulmonary venous saturation and systemic arterial saturations are equivalent, and the mixed venous saturation is best represented by the most distal saturation, typically the right or left branch pulmonary artery (PA) saturation. Thus in the absence of an intracardiac shunt, only two saturations are needed. Despite this, congenitally trained interventional cardiologists will always obtain saturations (sometimes several) from multiple locations throughout the heart and lungs to overcome potential sampling errors, which are particularly prevalent when there has been inadequate mixing of blood from various sources. Fig. 37.1 summarizes normal saturation ranges and demonstrates the expected range of sampling error at various locations throughout the heart. Sampling error is greatest in chambers receiving blood from multiple sources (e.g., the right atrium) and is lowest in the distal branch PAs due to more complete mixing of blood.

When there is a left-to-right shunt lesion (e.g., atrial septal defect [ASD] or ventricular septal defect [VSD]), there will be an increase (step-up) in saturations into the PAs reflecting the degree of shunting. In this scenario the pulmonary arterial saturation no longer accurately represents the mixed venous saturation. Instead the superior vena cava (SVC) saturation is typically used as the next best source for mixed venous saturation. In our opinion the SVC saturation is preferable to the inferior vena cava (IVC) saturation for estimating mixed venous saturation due to the wide variability in saturations in the IVC, which receives blood streaming from the low-saturation hepatic veins and from the higher-saturated renal circulation. When systemic desaturation is present, it is important to obtain saturations directly from the pulmonary veins to determine whether desaturation is from right-to-left shunting, pulmonary venous desaturation, or a combination of the two.
Assessment of Pressure Data
Pressure measurements on the right side of the heart are typically obtained in bilateral branch PAs, the main PA, right ventricle, and right atrium. On the left side of the heart, a pulmonary capillary wedge pressure (PCWP) may be used to estimate left atrial pressures (unless there is an ASD or patent foramen ovale permitting direct assessment), and direct pressure measurements are obtained in the left ventricle, ascending aorta, and descending aorta. Any pressure gradient reflects a degree of obstruction across the vascular structure being evaluated. Fig. 37.2 summarizes the normal range of pressures in the various chambers of the heart and in the lungs. Fig. 37.2 demonstrates normal intravascular waveforms, and Fig. 37.3 provides select examples of abnormal pressure tracings in patients with obstructive lesions. Typically peak systolic or “peak-to-peak” gradients are used to estimate stenosis of the semilunar valves or arterial vascular structures (e.g., aortic stenosis, coarctation of the aorta), whereas mean gradients are used to estimate venous or atrioventricular (AV) valve stenosis. When AV valve stenosis is being evaluated, an estimate of the severity of stenosis can be obtained by comparing the atrial a -wave and the ventricular end-diastolic pressure. However, the most accurate measure of AV valve stenosis is obtained by measuring the area under the curve for simultaneously obtained atrial pressures (or PCWP in place of the left atrial pressure) and ventricular pressures (see Fig. 37.3C ). When a patient is on the ventilator, pressure measurements are made at end expiration to minimize the effects of intrathoracic pressure on intracardiac pressures


Calculations of Flows
Pulmonary (Q p ) and systemic (Q s ) blood flow is estimated in the catheterization laboratory using the Fick method, which requires measuring the rate of change of an indicator through the heart or across a vascular bed. The two most common indicators are cold saline and oxygen content. When cold saline indicator is used, the approach is known as thermodilution and requires a specific catheter (e.g., thermodilution or Swan-Ganz catheter) with a proximal side hole and a distal thermistor. The side hole is positioned in the right atrium or a systemic vein while the thermistor is positioned in a branch PA. A known quantity of saline that is at least 10 degrees colder than the patient’s body temperature (typically 5 to 10 mL) is injected rapidly through the side hole into the right atrium. If cardiac output is high, then the cold saline mixes with a relatively larger amount of blood, and the temperature change at the thermistor is therefore relatively small. If cardiac output is low, then the cold saline mixes with a smaller quantity of blood, and the temperature change at the thermistor is more dramatic. The thermodilution technique is not accurate whenever there is disruption of flow, for example, if there is significant tricuspid valve regurgitation, pulmonary valve regurgitation, or an intracardiac shunt. As an alternative to cold saline, oxygen content can be used as the indicator. If one knows the rate at which oxygen is being added or extracted from the vascular bed (estimated by the patient’s volume of oxygen utilization [VO 2 ]), then flow can be estimated based upon the change in oxygen content. Table 37.1 lists some of the important equations that are used in the cardiac catheterization laboratory to calculate flows using the Fick principle.
VO 2 represents the rate of oxygen consumption by the body, which is equivalent to the rate of oxygen delivery by the lungs. VO 2 can be directly measured but is more commonly estimated using heart rate and age-dependent tables published in 1970 by Lafarge and Miettinen. Estimated VO 2 is a significant potential source of error in catheterization calculations. Rutledge and colleagues demonstrated greater than 50% error when VO 2 estimates were applied to mechanically ventilated children less than 3 years of age, and Seckeler and colleagues identified single-ventricle anatomy and critical illness as additional sources of substantial error. These limitations should be considered when evaluating cardiac catheterization data reliant upon VO 2 (e.g., Q p , Q s , and pulmonary vascular resistance [PVR]). Ratios such as Q p :Q s and pulmonary to systemic vascular resistance (PVR:SVR) eliminate VO 2 from the equation and are helpful in evaluating catheterization data. It is also important to consider the entirety of the data without placing excess emphasis on any single measured, estimated, or calculated number.
Estimating Shunts
The ratio of pulmonary to systemic blood flow (Q p :Q s ) is an important consideration in many congenital heart lesions. In a patient breathing room air (21% fraction of inspired O 2 [FiO 2 ]) Q p :Q s can be easily estimated using the following equation:
Q p : Q s = Systemic arterial saturation − mixed venous saturation Pulmonary venous saturation − pulmonary arterial saturation
This equation is not accurate when a patient is breathing a significantly higher FiO2 because it does not consider dissolved O 2 (i.e., O 2 that is not bound to hemoglobin). In room air, dissolved O 2 contributes relatively little to the oxygen content of blood and can be ignored, but this is not the case when a patient is breathing a higher FiO 2 . When a patient has bidirectional shunting, it is often helpful to estimate the magnitude of the right-to-left and left-to-right shunts in addition to the Q p :Q s ratio. These can be obtained by calculating the patient’s effective pulmonary blood flow, which represents the volume of desaturated blood flowing to the pulmonary vascular bed (i.e., excluding any left-to-right shunt), and the effective systemic blood flow, which represents the volume of fully saturated blood flowing to the systemic vascular bed (i.e., excluding any right-to left-shunt). Note from Table 37.1 that the equations for effective pulmonary and systemic blood flow estimation are identical. Fig. 37.4 documents catheterization data from a patient with transposition physiology, demonstrating a clinical scenario in which understanding effective pulmonary and systemic blood flow, as well as right-to-left and left-to-right shunting, may be useful. In this patient, and any patient with transposition physiology, the Q p :Q s is often high, but the effective pulmonary blood flow is low, leading to severe systemic desaturation.

Calculating Resistance
Resistance is calculated as the change in mean pressure divided by flow (see Table 37.1 ). The concept of resistance is particularly important in congenital heart disease because many shunt lesions can affect pulmonary or systemic pressures and blood flow. In patients with posttricuspid shunt lesions, PA pressures alone do not accurately reflect a patient’s operative risk, and the PVR is more relevant. Importantly, however, standard PVR calculations are unreliable when there is branch PA stenosis because the relative right- versus left-sided flow and pressures will differ. In this scenario PVR can be reliably estimated only if the relative flow distribution and pressure in each lung segment is known. Because the lungs represent resistors in parallel, each individual lung actually has a higher resistance value than the combined resistance of the two lungs together. This is evident from the equation for calculating total resistance when there are multiple resistors in parallel:
1 Total lung resistance = 1 Left lung resistance + 1 Right lung resistance
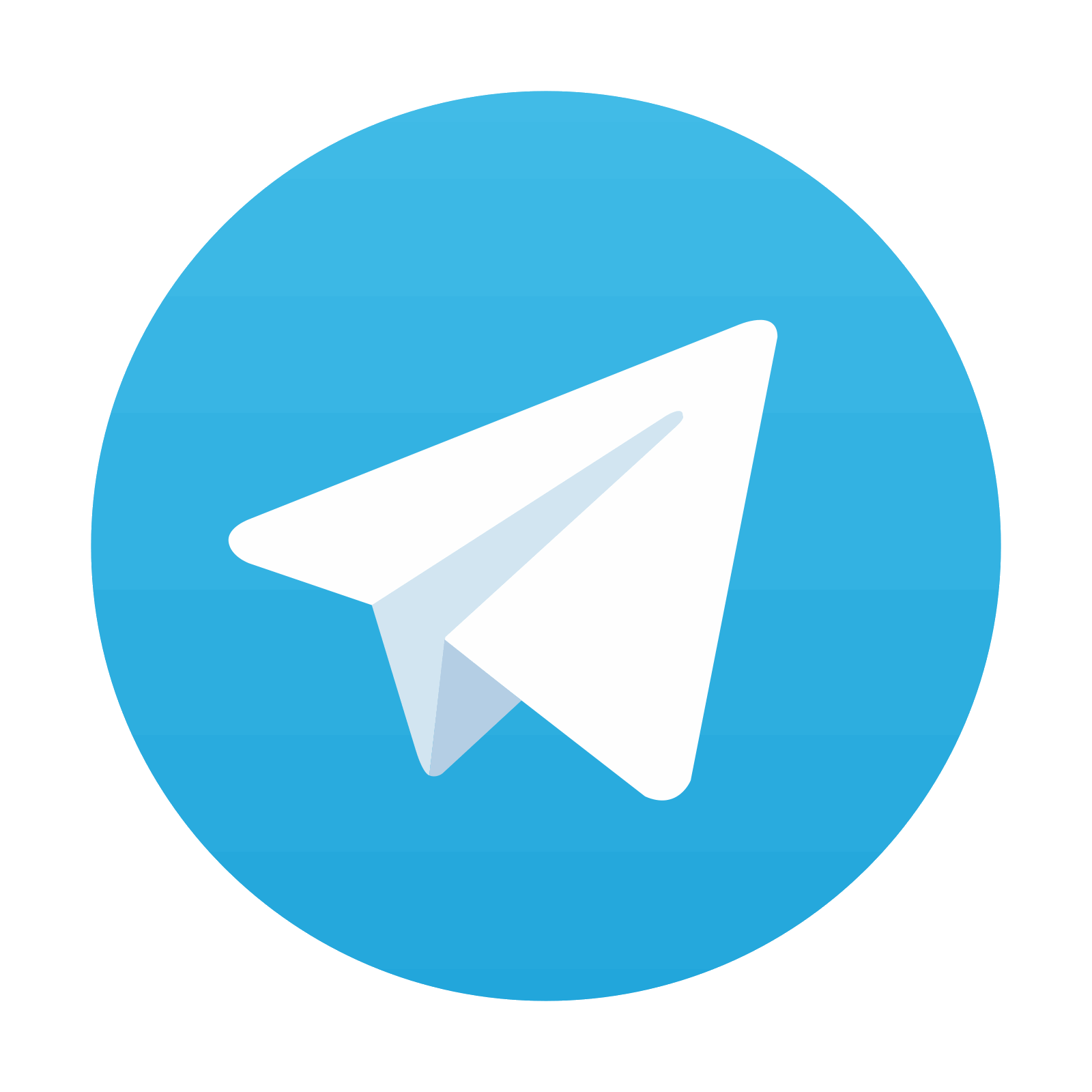
Stay updated, free articles. Join our Telegram channel
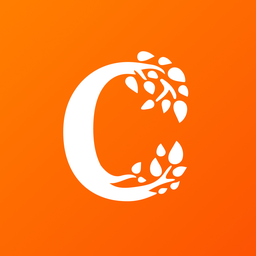
Full access? Get Clinical Tree
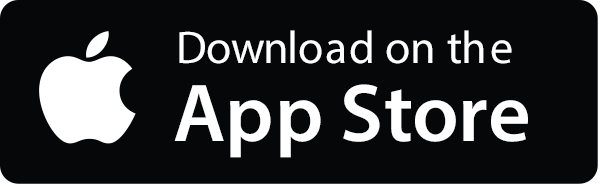
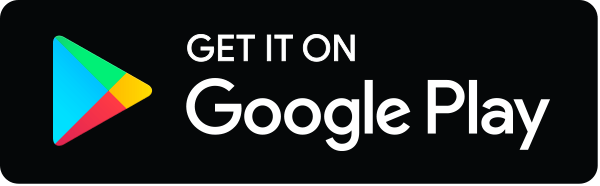