Ion
Inside (mM)
Outside (mM)
Na+
20
145
K+
150
4
Ca2+
0.0001
2.5
Cl−
25
140
To understand how concentration gradients of ions across a cell membrane affect membrane potential, consider a cell in which K+ is the only ion other than the large negatively charged proteins inside of the cell. In this cell, K+ diffuses down its chemical gradient out of the cell because its concentration is much higher inside than outside the cell. As K+ diffuses out of the cell, it leaves behind negatively charged proteins, thereby creating a separation of charge and a potential difference across the membrane (leaving it negative inside the cell).
The membrane potential that is necessary to oppose the movement of K+ down its concentration gradient is termed the equilibrium potential for K+ (EK) and is expressed by the Nernst potential. The Nernst potential for K+ at 37 °C is as follows:
where the potassium concentration inside [K+]i = 150 mM, and the potassium concentration outside [K+]o = 4 mM. The –61 is derived from RT/zF, in which R is the universal gas constant, z is the number of ion charges (z = 1 for K+; z = 2 for divalent ions such as Ca2+), F is Faraday’s constant and T is absolute temperature (°K). The equilibrium potential is the potential difference across the membrane required to maintain the concentration gradient across the membrane. In other words, the equilibrium potential for K+ represents the electrical potential necessary to keep K+ from diffusing down its chemical gradient and out of the cell. An increase in the outside K+ concentration will reduce the chemical gradient for diffusion out of the cell, i.e. the membrane potential required to maintain electrochemical equilibrium would be less negative according to the Nernst equation. The EM for a ventricular myocyte is about −90 mV, which is very close to the equilibrium potential for K+. Because the equilibrium potential for K+ is −96 mV and the resting membrane potential is −90 mV, a net driving force (net electrochemical force) acts on the K+, causing it to diffuse out of the cell. In the case of K+, this net electrochemical driving force is the EM (−90 mV) minus the EK (−96 mV), resulting in +6 mV. Because the resting cell has a finite permeability to K+ and a small net outward driving force is acting on K+, K+ slowly leaks outward from the cell.
![$$ {E}_K=-61\cdot \log \frac{{\left[{K}^{+}\right]}_i}{\left[{K}^{+}\right]}=-96 mV $$](/wp-content/uploads/2016/07/A321847_1_En_46_Chapter_Equa.gif)
The sodium ions play a major role in determining the membrane potential. Because the Na concentration is higher outside the cell, this ion would diffuse down its chemical gradient into the cell. To prevent this inward flux of Na, a large positive charge is needed inside the cell (relative to the outside) to balance out the chemical diffusion forces. This potential is called the equilibrium potential for Na+ (ENa) and is calculated using the Nernst equation, as follows:
where the sodium concentration inside [Na+]i = 20 mM, and the sodium concentration outside [Na+]o = 145 mM. The calculated equilibrium potential for sodium indicates that to balance the inward diffusion of Na+ at these intracellular and extracellular concentrations, the cell interior has to be +52 mV to prevent Na+ from diffusing into the cell.
![$$ {E}_{Na}=-61\cdot \log \frac{{\left[N{a}^{+}\right]}_i}{\left[N{a}^{+}\right]}=+52 mV $$](/wp-content/uploads/2016/07/A321847_1_En_46_Chapter_Equb.gif)
46.2.2 The Cardiac Conduction System
The conducting system of the heart consists of group of several cardiac muscle cells and conducting fibres, which are specialized for initiating impulses and conducting them rapidly through the heart (Fig. 46.1). They initiate the normal cardiac cycle and coordinate the contractions of cardiac chambers, first contract both atria, then the ventricles. The conducting system provides the heart its automatic rhythmic beat. For the heart to pump efficiently and the systemic and pulmonary circulations to operate in synchrony, the events in the cardiac cycle must be coordinated. The cardiac impulse originates in the sinoatrial node (SA node), located in the right atrium, which is activated first followed by the left atrium. The general direction of the atrial activation is inferiorly, to the left, and posteriorly. This causes the atria to contract and pump blood from the atria to the ventricles; it is recorded on an EKG as a P wave (Fig. 46.1). The atrial impulse is delayed in the atrioventricular node (AV node) to allow the ventricular chambers to fill and is then conducted rapidly through the ventricles (the bundle of His, the right and left bundles and the Purkinje fibres). This causes the ventricles to pump blood out of the heart and to the body; it is recorded on an EKG as a QRS complex. Recovery following the cardiac cycle, or repolarization, follows. This is recorded as a T wave on an EKG. On the microscopic level, the wave of depolarization propagates to adjacent cells via gap junctions located on the intercalated disc. The heart is a functional syncytium (not to be confused with a true “syncytium” in which all the cells are fused together, sharing the same plasma membrane as in skeletal muscle). In a functional syncytium, electrical impulses propagate freely between cells in every direction, so that the myocardium functions as a single contractile unit. This property allows rapid, synchronous depolarization of the myocardium. While advantageous under normal circumstances, this property can be detrimental, as it has potential to allow the propagation of incorrect electrical signals. These gap junctions can close to isolate damaged or dying tissue, as in a myocardial infarction.
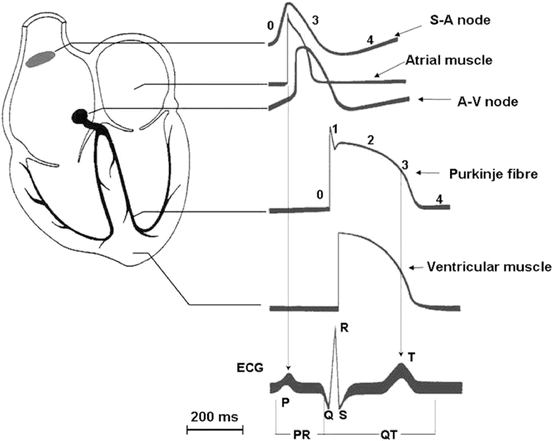
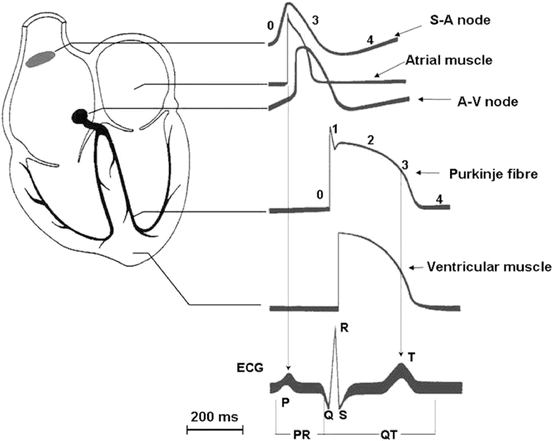
Fig. 46.1
Electrical activity in the myocardium. Schematic representation of a human heart with illustration of typical action potential (AP) waveforms recorded in different regions and their contribution to surface electrocardiogram
46.2.3 The Cardiac Action Potential
The normal mechanical (pump) function of the mammalian heart depends on proper electrical function [1, 2], as reflected in the successive activation of cells in specialized, “pacemaker” regions of the heart and the propagation of activity through the ventricles. Myocardial electrical activity is attributed to the generation of action potentials (AP) in individual cardiac cells, and the normal coordinated electrical functioning of the whole heart is readily detected in surface electrocardiograms (Fig. 46.1). Propagation of the electrical activity and coordination of the electromechanical functioning of the ventricles strongly depend on cellular electrical coupling mediated by gap junctions [3]. The generation of myocardial action potentials reflects the consecutive activation and inactivation of ion channels that conduct depolarizing, inward (Na+ and Ca2+), and repolarizing, outward (K+), currents. The waveforms of action potentials in different regions of the heart are different reflecting to differences in the expression and/or the properties of the underlying ion channels. These differences contribute to the normal unidirectional propagation of excitation through the myocardium and to the generation of normal cardiac rhythms [4–6].
The cardiac electrical cycle has been divided in to five “phases”, four of them describing the AP contour and one the diastolic interval (Figs. 46.1 and 46.2). Phase 0 refers to the autoregenerative depolarization, which occurs when the excitation threshold is exceeded. Phase 0 is supported by activation of two inward (depolarizing) currents, INa and ICaL. Membrane depolarization will quickly activate these channels and, with a delay of several milliseconds for INa and of tens of milliseconds for ICaL, inactivates them. Thus, membrane depolarization provides both the triggering and breaking mechanisms for the autoregenerative depolarization. Although short lived, INa is large and provides most of the charge influx required for AP propagation (see below). ICaL has a small component with fast activation/inactivation (ICaT) and a larger one with slower kinetics (ICaL). ICaL mediates most of Ca2+ influx required to trigger myocyte contraction and may support propagation under conditions in which INa is not expressed or functional (e.g. in the SA node). Phase 0 depolarization also activates K+ currents, which contribute to termination of this phase and to subsequent repolarization. Among these, the transient outward current (Ito) is fast enough to limit the depolarization rate during phase 0.
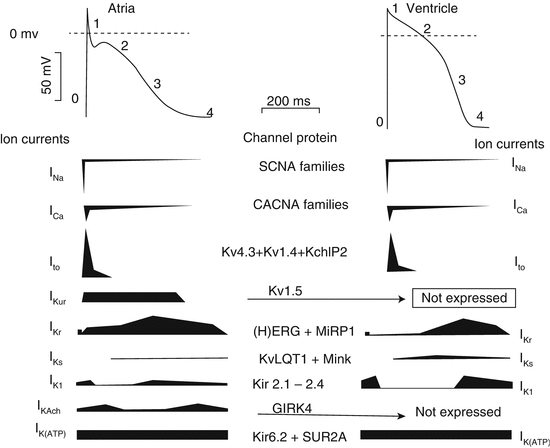
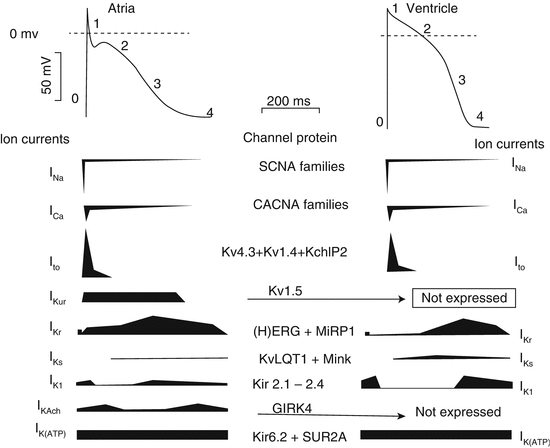
Fig. 46.2
Phases of a typical atrial and ventricular APs and underlying currents. The numbers refer to the five phases of the action potential. In each current profile, the horizontal line represents the zero-current level; inward currents are below the line, and outward currents are pointing upward
Phase 1 is the initial phase of repolarization, mainly supported by Ito,f (the Ca independent Ito fast component – see Sect. 46.3.3.1 for details), a potassium current that, similarly to INa, is activated and quickly inactivated by depolarization. Thus, Ito,f supports fast repolarization.
Phase 2, also named “plateau”, is the slow repolarization phase, which accounts for the peculiar configuration of the cardiac AP. The net transmembrane current flowing during phase 2 is small and it results from the algebraic summation of inward and outward components. The outward one (promoting repolarization) mainly consists of depolarization-activated K+ currents collectively named “delayed rectifiers” (IK). IK is actually the sum of rapid (IKr) and slow (IKs) components (and ultrarapid in atria, IKur), carried by separate channels with different properties and pharmacology [7]. The inward phase 2 currents (opposing repolarization) are mostly carried by “window” components of INa and especially of ICaL, which flow when membrane potential (Vm) is such that the activated state of these channels is not yet completely offset by the inactivation process. This inward calcium movement is through long-lasting (L-type) calcium channels that open when the membrane potential depolarizes to about −40 mV. L-type calcium channels are the major calcium channels in cardiac and vascular smooth muscles. They are opened by membrane depolarization (they are voltage operated) and remain open for a relatively long duration consequently causing the relative long plateau phase of AP (corresponding to the long ST segment of the ECG). The current generated by operation of the Na+/Ca2+ exchanger (INCX) can variably contribute to phase 2, according to the magnitude and course of the cytosolic Ca2+ transient and to the subsarcolemmal Na+ levels.
Phase 3 is the terminal phase of repolarization, which differs from phase 2 for its faster repolarization rate. Phase 3 is dominated by IKr and IK1, both characterized by a kinetic property, named “inward rectification” [8, 9], suitable to support autoregenerative processes [10]. Initiation of phase 3 is probably supported by IKr, with a threshold determined by the balance between its onset and waning of phase 2 inward currents. IK1 takes over during the final part of phase 3 and effectively “clamps” membrane potential back to its diastolic value [11].
Phase 4 describes membrane potential during diastole. In myocytes expressing a robust IK1 (e.g. atrial and ventricular myocytes), Vm is stabilized at a value close to the current reversal, and a significant current source is required to re-excite the cell. Under such conditions, even small inward currents may cause progressive depolarization, eventually leading to re-excitation (automatic behaviour) [12]. Besides the time-dependent currents, specific for each AP phase, time-independent (or “background”) currents may also contribute to the whole AP course. These mainly include the Na+/K+ pump current (INaK) and the Na+/Ca2+ exchanger current (INCX). Direction and magnitude of these currents during the various AP phases will be determined essentially by their electromotive force.
46.3 Transmembrane Ion Channels in the Heart
46.3.1 Voltage-Gated Na+ (NaV) Currents
Voltage-gated cardiac Na+ (NaV) channels open extremely rapidly (within 3 ms) on membrane depolarization and, in principle, determine alone the rapidly rising phases of the action potentials recorded in mammalian ventricular and atrial myocytes and in cardiac Purkinje fibres [13, 14]. Although the properties of the NaV channels expressed in different cardiac cells are alike, the biophysical and pharmacological properties of these channels are quite different from NaV channels expressed in other excitable cells (neurons or skeletal muscle). Cardiac NaV channels, for example, are remarkably insensitive to the NaV channel toxin tetrodotoxin (TTX). On membrane depolarization, cardiac NaV channels activate and inactivate very rapidly [15]. Figure 46.3 shows typical representative recordings from a single cell of INa in control and during superfusion with 10 and 30 μmol/L quinidine, a well-known sodium channel blocker Class IA antiarrhythmic drug [16]. The threshold for NaV channel activation is negative (approximately −55 mV), and the activation of these channels is strongly voltage dependent. Importantly, the inactivation is also voltage dependent and is so fast that cardiac NaV channels can undergo voltage-dependent inactivation without ever opening.
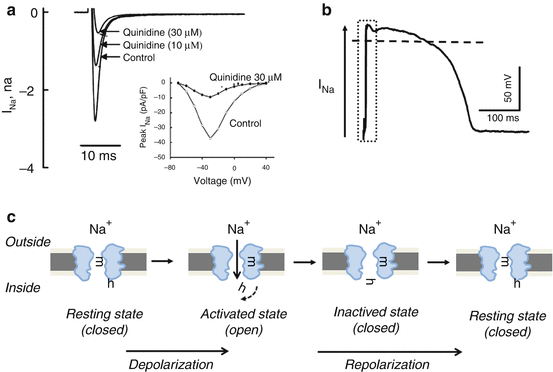
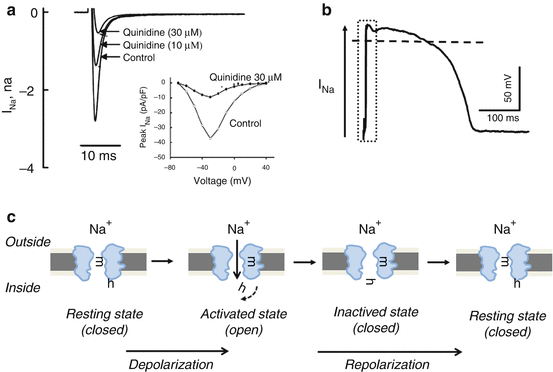
Fig. 46.3
The voltage-gated fast sodium channel (INa). (a) Original INa trace recorded in rabbit ventricular myocytes (Adapted from [16] with permission). The original recording shows representative recordings of peak INa from a single cell in the absence (control) and the presence of 10 and 30 μM/L quinidine (a typical Class IA, i.e. a known sodium channel blocker, antiarrhythmic drug). Inset shows current–voltage curves in the absence (open circles) and the presence (solid circles) of 30 μM/L quinidine. (b) The schematic contribution of INa to the phase 0 (depolarization) on the action potential shape. (c) The biophysical double-gating model of the sodium channel. Sodium channel has two gates, one activating gate (m) and one inactivating gate (h). In the resting (closed) state, the m-gates (activation gates) are closed, although the h-gates (inactivation gates) are open in order to wait activating pulse to open the channel. Rapid depolarization to threshold opens the m-gates (voltage activated), thereby opening the channel and enabling sodium to enter the cell. Shortly thereafter, as the cell begins to repolarize, the h-gates close and the channel becomes inactivated. Towards the end of repolarization, the m-gates again close, and the h-gates open. This brings the channel back to its resting state. Summarizing, the channel can be activated only after reaching again the resting phase (i.e. the activating gate must first close, and the inactivated gate must reopen). This phenomenon represents the subcellular basis of the refractoriness of the heart, i.e. the heart cannot be tetanized
At values corresponding to the action potential plateau in ventricular myocytes, present estimates are that 99 % of the NaV channels are already in an inactivated, nonconducting state [17]. There is, therefore, a finite, albeit small (1 %), probability of NaV channels being opened at potentials corresponding to the action potential plateau [17]. This slow component of NaV channel inactivation called late Na current (INaL) has indeed been described in normal human ventricular myocytes [18].
The probability of NaV channel opening at depolarized potentials (i.e. during phase 2) is determined by the overlap of the curves describing the voltage dependences of channel activation and inactivation. Consistent with these predictions, electrophysiological studies reveal the presence of a sustained component of inward NaV current, i.e. a “persistent” NaV current, during prolonged membrane depolarizations, which was named “window” current. Although the “window” current is relatively small in comparison with the magnitude of the INa current, this current may contribute to action potential durations [19]. It has been reported that the expression level of the persistent NaV current component is different in various regions of the ventricles, differences that could contribute to the observed regional heterogeneities in ventricular action potential durations [20]. The impact of alterations in the “persistent” NaV channel window current on cardiac rhythms has now been unequivocally demonstrated with the identification of mutations in the gene, SCN5A, encoding the TTX-insensitive cardiac NaV channels in patients with an inherited form of long QT syndrome, LQT3 [21]. Several SCN5A mutations in different affected individuals/families have been identified and linked to Brugada syndrome and to conduction defects, in addition to LQT3 [22, 23] (Chaps. 49 and 52).
46.3.2 Voltage-Gated Ca2+ (CaV) Currents
In contrast to skeletal muscle, it has long been recognized that Ca2+ entry from the extracellular space is required for excitation–contraction coupling in the mammalian myocardium [2, 24]. Several studies reported late that a “slow inward” (in comparison with NaV channel) current is carried by Ca2+ through a membrane conductance distinct from the Na+ movement [25]. Further studies characterized the time- and voltage-dependent properties of voltage-gated cardiac Ca2+ (CaV) currents in multicellular preparations and, later in isolated single cardiac cells as well [26, 27].
Based on differences in the thresholds for channel activation, two different types of CaV currents/channels were reported to be present in chick and rat sensory neurons [28, 29]. These channels were termed high-voltage-activated (HVA) and low-voltage-activated (LVA) CaV channels, respectively. In the heart, HVA and LVA CaV channels were first described in isolated canine atrial cells [30]. LVA CaV channels, also referred to as T-type Ca2+ channels [31], activate at relatively hyperpolarized membrane potentials, to about −50 mV, and these channels activate and inactivate rapidly [28, 29]. On the contrary, HVA CaV channels (L type) open on depolarization to more positive membrane potentials (approximately −20 mV) and inactivate over a longer time scale (several tens of milliseconds to seconds). Under physiological conditions, with Ca2+ as the charge carrier, HVA channels in most cells inactivate in <100 ms at depolarized voltages [30]. Figure 46.4 shows typical representative recordings from a dog ventricular single cell of ICaL in control and during superfusion with 10 μM/L dronedarone, an antiarrhythmic drug known to possess strong ICaL channel blocker effect [32].
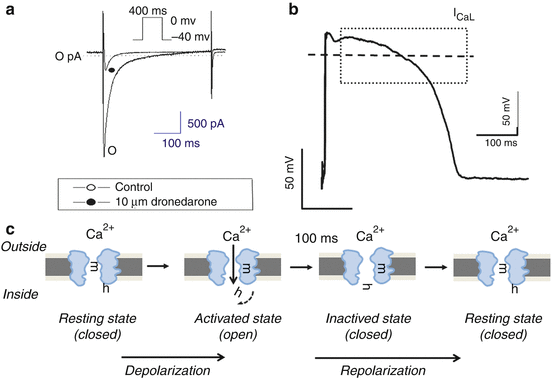
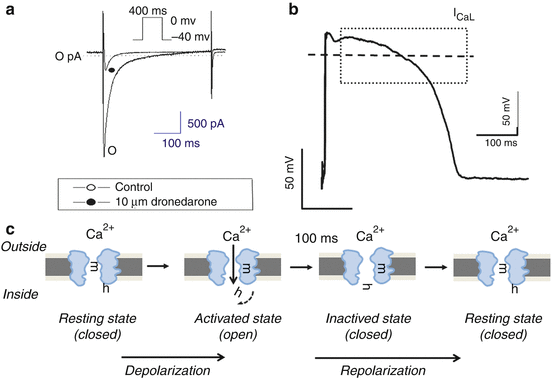
Fig. 46.4
The voltage-gated L-type calcium channel (ICaL). (a) Original ICaL trace recorded in dog ventricular myocytes (Modified from [32] with permission). The original recording shows representative recordings of ICaL from a single cell in the absence (control) and the presence of 10 μM/L dronedarone (known as iodine free “amiodarone” a typical multichannel antiarrhythmic drug, which is similar to amiodarone and a strong ICaL channel blocker). (b) The schematic contribution of ICaL to the phase 2 (depolarization) on the action potential shape. Lower panels (c) The biophysical double-gating model of the calcium channel. Calcium channels, like sodium channel, have two gates, one activating gate (m) and one inactivating gate (h). In the resting phase (left panel), m-gate is closed, while h-gate is open waiting for the activating pulse to open the channel. When membrane reaches the activating voltage threshold, m-gate opens facilitating the entrance of the Ca2+ ion from the extracellular space into the cell (2nd panel). The resulting inward current is extremely fast activating (within 1–3 s, see panel a, left). The inactivating gate (h) fast closes (3rd panel) causing the relative fast inactivation (to about 50–150 ms) of the ICaL current. This medium fast-inactivating calcium current is responsible for the relative long plateau phase of the AP shape. The double-gated cardiac calcium channel has the intrinsic properties that it cannot be reactivated again from the inactive state to open the channel. The channel can be activated only after reaching the resting phase (4th right panel) (i.e. the activating m-gate must first close and h the inactivated gate must reopen)
In the mammalian heart, L-type CaV channel currents appear to be ubiquitously expressed. Apparently, there are no interspecies and transregional differences in the properties, and the densities of L-type CaV channel currents occur, suggesting similar molecular compositions of the underlying channel. Since the opening of cardiac L-type CaV channels in response to membrane depolarization is delayed relative to the NaV channels, ICaL contributes little to phase 0 depolarization in Purkinje, atrial and ventricular cells (Figs. 46.1 and 46.2). The opening of L-type CaV channels and the Ca2+ entry through these channels are responsible for the action potential plateau (phase 2), which is particularly prominent in ventricular and Purkinje cells.
In addition, Ca2+ influx through the L-type CaV channels triggers Ca2+ release from intracellular Ca2+ stores and underlies excitation–contraction coupling in the working (ventricular) myocardium [1, 2, 33]. L-type CaV channels are also expressed in other heart regions as SAN and AVN cells, where they play an important role in action potential generation and automaticity regulation too [34]. Cardiac L-type CaV channels undergo rapid voltage- and Ca2+-dependent inactivation [35], processes that will also influence the action potential waveforms (Fig. 46.2) by interfering the duration of the plateau (phase 2) and the time course of action potential repolarization.
46.3.3 Voltage-Gated K+ Channels
Voltage-gated K+ (KV) channels are the primary determinants of action potential repolarization in the mammalian myocardium. There is considerable electrophysiological and functional cardiac KV channel diversity as compared with cardiac NaV and CaV channels [36]. Based primarily on differences in time- and voltage-dependent properties and pharmacological sensitivities [6, 36], two large classes of repolarizing cardiac KV currents have been distinguished: transient outward K+ currents (Ito) and delayed, outwardly rectifying K+ currents (IK). Ito currents activate and inactivate rapidly on membrane depolarizations to potentials positive to approximately −30 mV and underlie the early phase (phase 1) of repolarization (notch) in ventricular and atrial cells (Fig. 46.2). The cardiac delayed rectifiers (IK) activate at relative similar membrane potentials, however, having variable kinetics. IK currents determine the latter phase (phase 3) of repolarization back to the diastolic potential. Multiple types of myocardial Ito and IK channels were identified in various species. The distinct time- and voltage-dependent properties and differences in the densities and the biophysical properties of these channels contribute to variations in the waveforms of action potentials recorded in different cardiac cell types [4, 36] (see also Chaps. 49, 50 and 52).
46.3.3.1 Transient Outward KV Currents
Although cardiac transient outward currents were first described in sheep Purkinje fibres and thought to reflect Cl− conductance [37], subsequent work demonstrated the presence of two transient outward currents with distinct properties, and they were referred to as Ito1 and Ito2 [38]. Pharmacological studies revealed that the K+ selective Ito1 is blocked by high concentration of 4-aminopyridine (3–5 mM 4-AP) and is not affected by changes in extracellular Ca2+, whereas Ito2 cannot be blocked by 4-AP and is Ca2+ dependent [38].
In further studies, it was shown that the Ca2+-dependent Ito2 in Purkinje fibres and ventricular cells is a Cl− channel (Ca2+-activated chloride channel) [39]. The transient outward K+ currents, referred to variably by different laboratories as Ito, or Ito1 [40], have now been described in many cardiac cell types and in most species [41]. Comparison of the detailed biophysical properties of the transient outward K+ currents described in various cell types and species, however, suggested that there are two types of transient outward K+ currents (Fig. 46.5) [42]. This hypothesis is supported by several electrophysiological and pharmacological studies. In adult mouse and rat ventricular myocytes, for example, two transient K+ currents, termed Ito,fast (Ito,f) and Ito,slow (Ito,s), have been distinguished [43]. On membrane depolarization, mouse ventricular Ito,f channels activate and inactivate rapidly, and on membrane repolarization, these (Ito,f) channels recover rapidly from steady-state inactivation. Similar to Ito,f, mouse ventricular Ito,s channels activate and inactivate rapidly. Although the properties of the transient K+ currents in different cell types and species are similar and are amenable to classification as either Ito,f or Ito,s, there are large cell type and interspecies variations in the detailed biophysical properties of the (Ito,f and Ito,s) currents [41]. These observations suggest that there may well be subtle, albeit potentially important, cell type- and/or species-dependent molecular heterogeneity among Ito,f and Ito,s channels in different cell types and/or in different species.
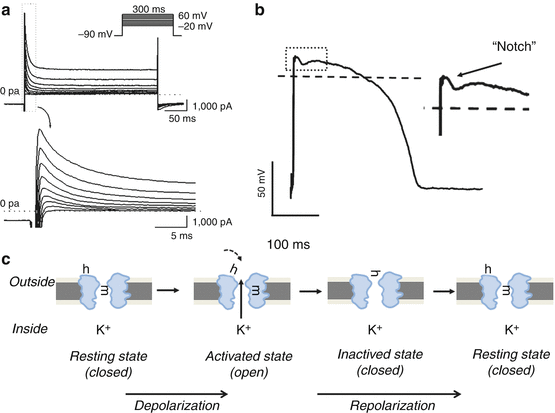
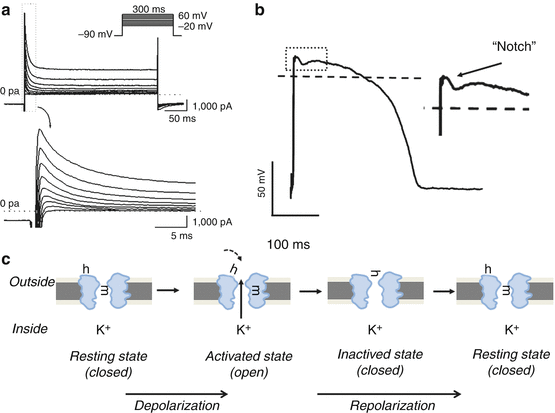
Fig. 46.5
The transient outward K+ channel (Itof). (a) Original Itof trace recorded in dog ventricular myocytes (This panel is modified from [42] with permission). Ito current was activated by 300 ms long depolarizing voltage pulses (inset top panel shows applied voltage pulse), while bottom panel shows the magnified Ito current recorded under the first 20 s long depolarizing pulse (corresponding with the magnification of Ito marked by the dashed-line rectangle on the top panel). (b) The schematic contribution of Ito,f to the phase 1 (early repolarization, the notch phase of the AP) on the action potential shape. (c) The biophysical double-gating model of the transient outward channel. Like fast sodium (see Fig. 46.3) and inward calcium (see Fig. 46.4) channels, Ito channels have two gates, one activating gate (m) and one inactivating gate (h). In the resting phase (left panel) m-gate is closed, while h-gate is open waiting the activating pulse to open the channel. When membrane potential reaches the activating voltage threshold, m-gate opens facilitating the exit of the K+ ion from the intracellular space to the extracellular space (2nd panel). The resulting outward current is extremely fast activating (within 1–3 s, see panel a, left). The inactivating gate (h) fast closes (3rd panel) causing the relative fast inactivation (to about 50–150 ms) of the Ito current. The double-gated cardiac Ito channel has the intrinsic properties that it cannot be reactivated again from the inactive state. The channel can be activated only after reaching again the resting phase (4th panel) (i.e. the activating gate must first close, and the inactivated gate must reopen)
Although originally identified in Purkinje fibres, Ito,f is a prominent repolarizing current in atrial and ventricular myocytes in the other species including humans [44–46]. In humans and other larger mammals (as dog), Ito,f underlies the early phase (phase 1, notch) of repolarization in ventricular and atrial cells (Fig. 46.5) and likely also contributes to determining the plateau (phase 2) [32, 41, 47, 48]. Recent studies reported that the transient outward potassium current Ito,f may influence not only indirectly by modulating the plateau duration but also directly the phase 3 of the ventricular repolarization in dog and human hearts [32, 49]. However, there are exceptions. In guinea pig ventricular cells, for example, Ito,f has not been detected except when extracellular Ca2+ is removed [50]. These channels are absent in rabbit atrial and ventricular cells [46]. Nevertheless, there are transient KV currents in rabbit myocytes (typically referred to as transient inward current It), which inactivate slowly and recover from (steady state) inactivation very slowly, whose properties more closely resemble mouse ventricular Ito,s than Ito,f and reflect possible different molecular structure background.
Transient KV currents classified as Ito,f have also been shown to be expressed in (rabbit) SAN cells, although, similar to NaV currents, Ito,f densities vary markedly among SAN cells. In addition, when expressed, Ito,f appears to play a role in shaping action potential waveforms and in regulating automaticity in SAN cells [51]. Cells isolated from the (rabbit) AVN also express Ito,f, and detailed kinetic analysis of the currents reveals the presence of two components with distinct rates of inactivation and recovery [52]. It is unclear whether these findings reflect differences in the kinetic properties of a single type of Ito,f channel or if two distinct types of Ito channels are expressed in (rabbit) AVN cells.
46.3.3.2 Delayed Rectifier KV Currents
The delayed rectifier potassium current (IK) is a major outward current responsible for ventricular muscle action potential repolarization [53]. This current was first described in 1969 by Noble and Tsien using the two-microelectrode voltage-clamp technique in sheep cardiac Purkinje fibre strands [54]. Since its discovery, it has been examined in single isolated myocytes obtained from various regions of the heart in several mammalian species [7, 55, 56]. In most species, IK can be separated into rapid (IKr) and slow (IKs) components (Fig. 46.6) that differ from one another in terms of their sensitivity to drugs, rectification characteristics and kinetic properties [55, 57–59].
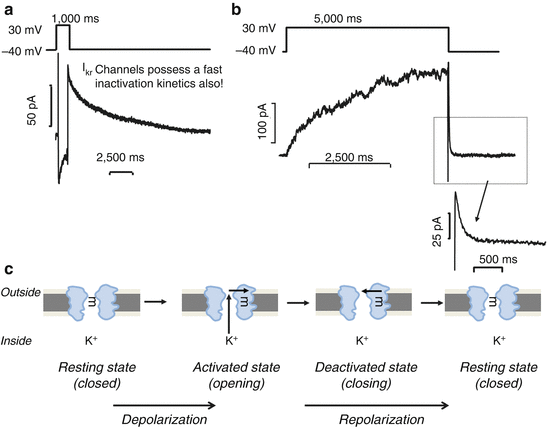
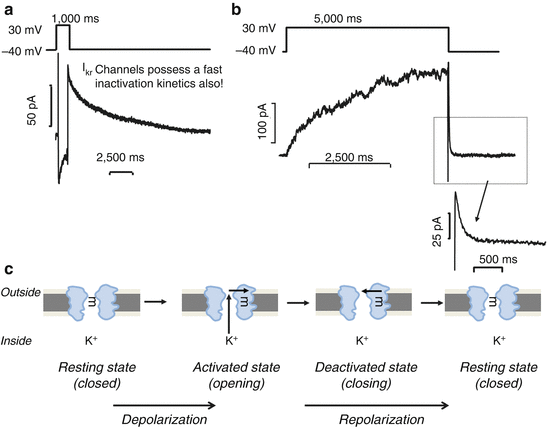
Fig. 46.6
The fast and the slow components of the delayed rectifier K+ channel (IKr and IKs). Upper panels. Original IKr (a) and IKs (b) traces recorded in human ventricular myocytes (Modified from [58, 59] with permission). IKr and IKs currents were activated using test pulses of 1,000 ms (IKr) or 5,000 ms (IKs) in duration to 20 mV from the holding potential of −40 mV. The decaying tail current at −40 mV after the test pulse was assessed as IKr and IKs tail currents. (c) The biophysical single-gating model of the delayed rectifier potassium channels. IK channels unlike the previously presented INa, ICa and Ito channels (see Fig. 46.3, 46.4 and 46.5, respectively) have only one channel gate, which in resting state is closed (left panel). When cells are depolarized, the channel activates (2nd panel). In IKr channels, this activation is fast, while in IKs channels it is relatively slow. When cells are repolarized, the channels are closed (3rd and 4th panels). IKs channels are not inactivating, thereby the closing mechanism is called deactivation. Previously it was thought that IKr behaves a similar way that IKs having one gate-based activating and deactivating kinetics, until Spector et al. [60] reported that IKr channels have in fact double-gating mechanism like Ito channels. IKr channels inactivate having extremely fast inactivation kinetics; however, this inactivation displays a voltage-dependent behaviour. At positive membrane potential, the inactivation is blocked, while when membrane potential repolarizes and becomes more negative; the inactivation blocking effect disappears, and channels deactivate with a relative slow kinetics
Although IKr activates rapidly, inactivates very rapidly and thereby displays marked inward rectification, no inward rectification is evident for the slowly activating IKs [60]. In cardiac ventricular myocytes isolated from healthy human donor hearts, IKr activated fast and deactivated slowly and biexponentially (τ 1 ≈ 600 ms and τ 2 ≈ 6,700 ms) [61], while IKs exhibited slow- and voltage-independent activation at more positive than 0 mV (τ ≈ 900 ms) and had fast and monoexponential deactivation kinetics [58, 59]. This deactivation was voltage dependent, being fast at more negative (at −50 mV, τ ≈ 90 ms) and slow at more positive voltages (at 0 mV, τ ≈ 350 ms) [59]. In dog cardiac ventricular cells, IKr activated fast and deactivated slowly and biexponentially (τ 1 ≈ 360 ms and τ 2 ≈ 3,300 ms), while IKs activated slowly (τ ≈ 800 ms) at voltage more positive than 0 mV and deactivated rapidly and monoexponentially (τ ≈ 150 ms) [55, 62]. In the guinea pig, where it was first described, IKs activated very slowly, not saturating even after 5–7 s at +50 mV, and deactivated slowly, within 500–1,000 ms [63]. Initially, it was not observed in the rabbit, but later studies revealed a large and consistent IKs in the rabbit ventricle [56, 64]. Considering the kinetic properties, the human cardiac slow delayed rectifier potassium current best resembles those measured in the dog [55, 62] and rabbit [56, 64] ventricle but significantly differs from those found in the guinea pig heart [57]. IKs was augmented with an increase in sympathetic tone as a result of elevation in intracellular cAMP levels [58, 65].
< div class='tao-gold-member'>
Only gold members can continue reading. Log In or Register a > to continue
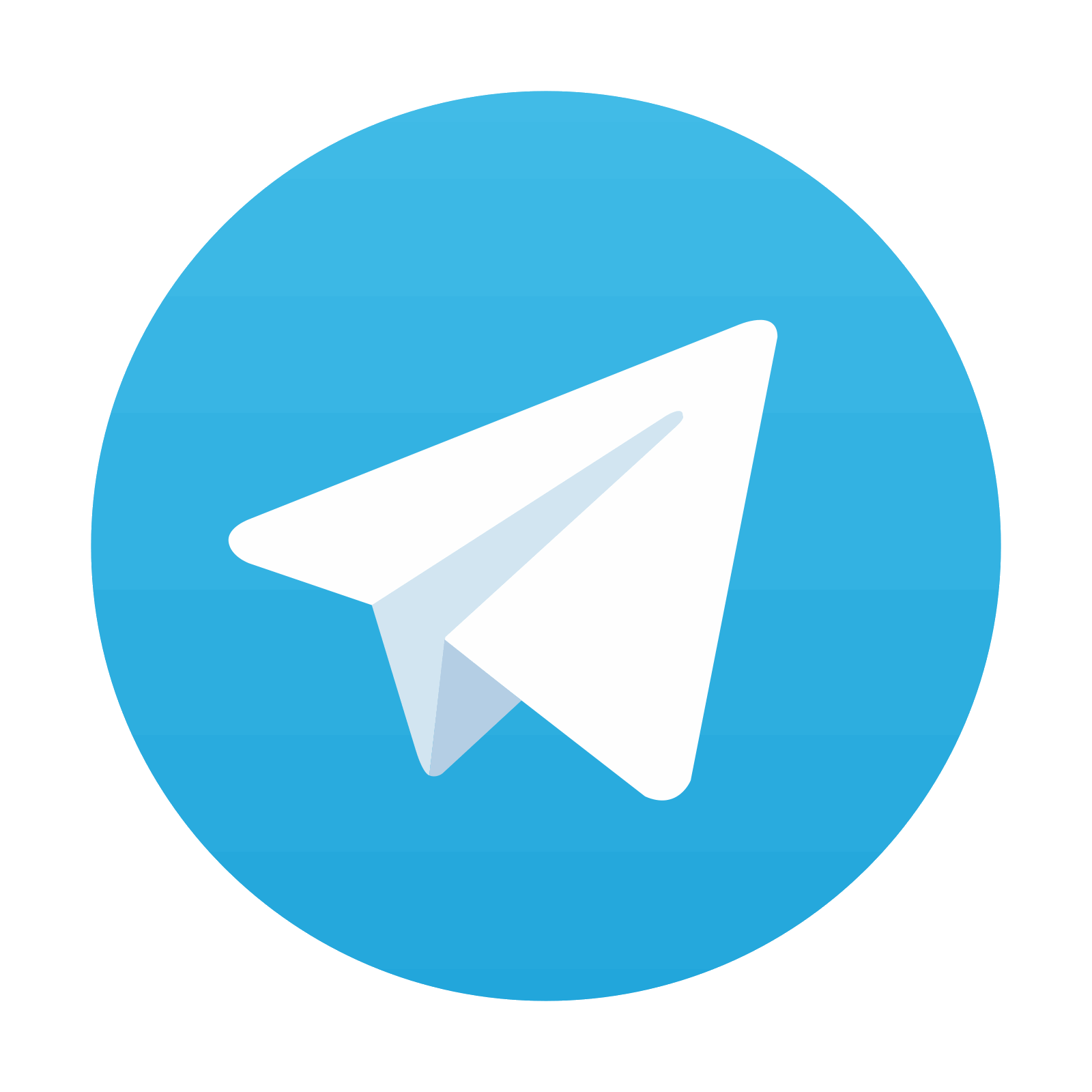
Stay updated, free articles. Join our Telegram channel
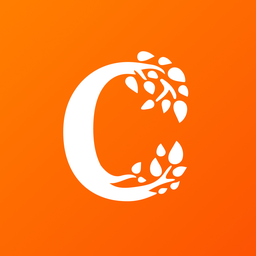
Full access? Get Clinical Tree
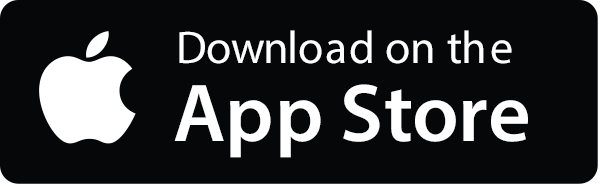
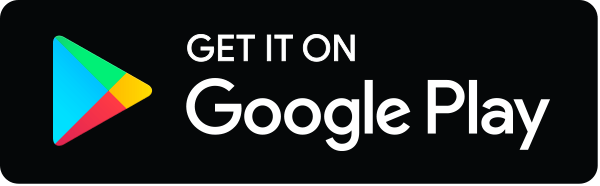