ST segment abnormalities in leads V1–V3
Type 1
Type 2
Type 3
J-point
≥2 mm
≥2 mm
≥2 mm
T-wave
Negative or isoelectric
Positive or biphasic
Positive
ST-T configuration
Coved type
Saddleback
Saddleback
ST segment (terminal portion)
Gradually descending
Elevated ≥1 mm
Elevated <1 mm
Diagnosis of Brugada syndrome is also considered positive when a Type 2 (saddleback pattern) or Type 3 ST-segment elevation is observed in more than one right precordial lead under baseline conditions and can be converted to the diagnostic Type 1 pattern upon exposure to sodium channel blocker (ST-segment elevation should be ≥2 mm). One or more of the clinical criteria described above should also be present. Drug-induced conversion of Type 3 to Type 2 ST-segment elevation is considered inconclusive for diagnosis of Brugada syndrome.
The ECG manifestations of the syndrome are often concealed, but can be unmasked using sodium channel blockers. Definitive diagnosis is difficult when the degree of basal ST-segment elevation is relatively small and the specificity of sodium channel blockers such as flecainide, ajmaline, procainamide, disopyramide, propafenone and pilsicainide [22, 31, 32] to identify patients at risk is uncertain. A comparison of intravenous ajmaline and flecainide in the same cohort of patients revealed that ajmaline is more effective in unmasking the syndrome [33]. Flecainide failed in 7 of 22 cases (32 %) unmasked by ajmaline. A greater inhibition of transient outward current (Ito) by flecainide renders it less effective than ajmaline. False positive as well as false negative responses have been reported with ajmaline, as well, although a false positive is difficult to prove [34, 35]. Itoh et al. demonstrated that a specific missense mutation in SCN5A, the gene that encodes the α subunit of the sodium channel, although responsible for the disease, prevented the ability of ajmaline to unmask the syndrome, due to loss of the ability of the drug to produce use-dependent inhibition of sodium channel current [35].
Most cases of Brugada syndrome display right precordial ST-segment elevation, although isolated cases of inferior lead [36, 37] or left precordial lead [38] ST-segment elevation have been reported in Brugada-like syndromes, in some cases associated with SCN5A mutations. In rare cases, ST segment elevation is observed in all precordial leads. The appearance of an early repolarization pattern, characterized by J point elevation, distinct J waves, slurring of the terminal part of the QRS and/or ST segment elevation, in the inferior or infero-lateral leads in patients with Type I ST segment elevation in the anterior leads has been associated with adverse prognosis [39–41]. This type of global ST segment elevation, manifesting in inferior, lateral and anterior leads, has been termed Type 3 Early Repolarization Syndrome (ERS) in a recent proposed classification of ERS [42].
Placement of the right precordial leads in a superior position (up to the second intercostal spaces above normal) can increase the sensitivity of the ECG for detecting the Brugada phenotype in some patients, both in the presence or absence of a drug challenge [43, 44] or during Holter recordings [45]. Studies are underway to ascertain whether the greater sensitivity is at the cost of a lower specificity and whether a Type I ECG in the elevated leads is as predictive of events as a Type I ECG the in the standard leads. A recent report demonstrates that as many as 1.3 % of normal Korean males display a Type 2, but not Type 1, ST segment elevation when the right precordial leads are recorded from a superior position [46].
A slight prolongation of the QT-interval is sometimes observed associated with the ST segment elevation [32, 47, 48]. The QT-interval is prolonged more in the right vs. left precordial leads, presumably due to a preferential prolongation of action potential duration (APD) in right ventricular (RV) epicardium secondary to accentuation of the action potential notch [49]. A QTc >460 ms in V2 has been shown to be associated with arrhythmic risk [50]. Depolarization abnormalities including prolongation of P-wave duration, PR and QRS intervals are frequently observed, particularly in patients linked to SCN5A mutations [51]. PR prolongation likely reflects HV conduction delay [47]. QRS fragmentation, defined as three or more spikes in the QRS complex, has been found to be an independent predictor of spontaneous arrhythmic events [52, 53].
In many cases arrhythmia initiation is bradycardia-related [54]. This may contribute to the higher incidence of sudden death at night in individuals with the syndrome and may account for the success of pacing in controlling the arrhythmia in isolated cases of the syndrome [55]. Makiyama and co-workers reported that loss-of-function SCN5A mutations resulting in Brugada syndrome are distinguished by profound bradyarrhythmias [56]. Related to this observation is the recent report by Scornik and co-workers [57] demonstrating expression of the cardiac sodium channel gene, SCN5A, in intracardiac ganglia. This interesting finding suggests that a loss of function mutations in SCN5A may not only create the substrate for reentry in ventricular myocardium, but may also increase vagal activity in intracardiac ganglia, thus facilitating the development of arrhythmias in patients with the Brugada syndrome. This finding may also explain the frequent association of vasovagal syncope with BrS [58].
A polymorphic ventricular tachycardia (VT) is most commonly associated with the Brugada syndrome. Monomorphic ventricular tachycardia (VT) is observed infrequently and is generally more prevalent in children and infants [59–64].
The majority of congenital Brugada syndrome patients are believed to possess a structurally normal heart, consistent with the notion that this is a primary electrical heart disease [65]. While fibrosis and myocarditis may exacerbate or indeed trigger events in patients with the Brugada syndrome, it seems clear that in the vast majority of cases these structural changes are unrelated to arrhythmogenic right ventricular cardiomyopathy/dysplasia (ARVC/D).
Prognosis and Risk Stratification
Risk stratification of patients with the Brugada syndrome has been an issue of lively debate. It is generally accepted that Brugada syndrome patients presenting with aborted sudden death are at high risk for recurrence and that they should be protected by an implantable cardiac defibrillator (ICD). There is also little argument that a patient with Brugada syndrome presenting with syncope, particularly when the clinical history suggests an arrhythmic syncope (as opposed to typical vasovagal syncope), are at high risk. In contrast, risk stratification of asymptomatic patients has met with considerable debate [11, 15, 66–70]. Several invasive and noninvasive parameters have been proposed for identification of patients at risk of sudden death, including the presence of spontaneous Type 1 ST segment elevation, the characteristics of the S wave [71], wider QRS complexes [72], the presence of late potentials [73] or QRS fragmentation [52, 53] and inducibility of VT/VF using programmed electrical stimulation (PES) [74].
In 1998, Brugada et al. [75] reported that over a 34 month follow-up period, 27 % of previously asymptomatic patients experienced a first ventricular fibrillation or sudden cardiac death. This figure corresponds to an occurrence of life-threatening events of approximately 10 % per year. In 2002, with a mean follow-up of 27 ± 29 months, the same authors [66] reported that 8 % of previously asymptomatic patients had become symptomatic; an occurrence of a life-threatening event of 3.5 % per year. In 2005, Brugada et al. [68] reported that 6 % of asymptomatic patients displayed a first event during a mean follow-up of 42 ± 42 months, corresponding to an event rate of 1.7 % per year. This progressive decline in first event rate in previously asymptomatic patients most likely reflects a reduced severity of phenotypes referred to the Brugada registry in subsequent years. In contrast, Priori et al. in 2002 [67] reported that asymptomatic patients have a cumulative probability of 14 % for developing a cardiac arrest by age 40, corresponding to an natural history incidence of cardiac arrest of 0.35 % per year. In 2005, the same authors reported a first event rate of 3 % (4/132) over a 31 month follow-up period, corresponding to an event rate of 1 % per year [69].
The reason behind the disparity in the data generated by these two groups is not clearly evident. It was suggested by Brugada et al. [68] that the difference may be due to the inclusion by Priori and co-workers of patients with Type 2 and 3 ST segment elevation, which is not considered diagnostic of the Brugada syndrome [7–10]. Priori and co-workers argue that exclusion of Type 2 and 3 from the diagnosis of the syndrome can lead to missed diagnosis of the disease [69]. While this is clearly the case, it may be a rare occurrence, and the exclusion appears justified on the basis that it avoids a large number of false positive diagnoses. As a result of the failure to exclude individuals with Type 2 and 3 ST segment elevation, the European registry may contain many individuals who do not have the syndrome. However, a recent report by Eckardt and co-workers [70] suggests that other factors may be involved. These authors report that 1 out of 123 asymptomatic individuals with a Type 1 ECG (0.8 %) had a first arrhythmic event during a 40 ± 50 month follow-up. This translates into a first event rate of 0.24 % per year, considerably less than the other two registries. Thus, as additional data have become available, it has become clear that the prognosis of asymptomatic patients is associated with much less risk than initially perceived.
The large registry studies agree that Brugada syndrome patients at higher risk for the development of subsequent events are those presenting with a spontaneous Type 1 ST segment elevation or Brugada ECG and/or those with a previous VT/VF or Sudden Cardiac Death (SCD) [70]. The registries also agree that PES inducibility is greatest among patients with previous VT/VF or syncope. Approximately 1/3 of asymptomatic patients are inducible. In the Priori et al. [69] and Eckardt et al. [70] studies inducibility of VT/VF in asymptomatic patients was not associated with risk. The lack of association between inducibility and spontaneous VF in Brugada patients was also reported by a number of smaller studies, such as that of Kanda et al. [76].
In sharp contrast, Brugada et al. [77] found that the risk for developing VT/VF is considerably greater in patients who are inducible during PES, whether or not a Type 1 ST segment elevation was spontaneously present or whether or not they were symptomatic. The reason for the marked disparity in the predictive power of PES inducibility among the different studies is not immediately apparent. The discrepancies may be due to differences in patient characteristics and the use of multiple testing centers with non-standardized or non-comparable stimulation protocols [78]. Additional studies are clearly needed to further define risk stratification strategies for asymptomatic patients.
It is noteworthy that in experimental models of the Brugada syndrome involving the coronary-perfused wedge preparation, polymorphic VT is readily inducible with a single ventricular extrastimulus, but only when applied on the epicardial surface of the wedge. Inducibility is not possible or much more difficult when extra-stimulation is applied to the endocardial surface. The shorter refractory period of epicardium allows extra-stimuli direct access to the vulnerable window across the ventricular wall, thus facilitating the induction of reentry. These relationships suggest that PES applied to the epicardium may provide a more accurate assessment of risk than the current clinical approach in which stimuli are applied to the endocardial surface. In support of this hypothesis, Carlsson et al. reported that a Brugada syndrome patient with recurrent syncope due to polymorphic ventricular tachycardia could not be induced with right ventricular endocardial stimulation. However, epicardial stimulation from a left ventricular site through the coronary sinus led to the development of polymorphic VT [79].
Gehi et al. [80] recently reported the results of a meta-analysis of 30 prospective studies that included 1,545 patients with a Brugada ECG to assess predictors of events. The meta-analysis suggested that a history of syncope or SCD, the presence of a spontaneous Type I Brugada ECG, and male gender predict a more malignant natural history. The findings however did not support the use of a family history of SCD, the presence of an SCN5A gene mutation, or electrophysiologic study to guide the management of patients with a Brugada ECG. A similar meta-analysis by Paul et al. [81] also concluded that the inducibility of VF in asymptomatic patients is of no prognostic value.
Since the publication of the meta-analyses by Gehi et al. and Paul et al., two large multicenter studies from Japan [82] and from Europe (the FINGER study) [83] were published. The Japanese study is important because it included only probands (330 probands of whom 154 were asymptomatic) and the FINGER study was important because the number of asymptomatic patients included (n = 654) was significantly larger than the number of asymptomatic patients included in the last published series by Brugada (n = 263) and even larger than the 457 patients number compiled from 15 studies in the meta-analysis by Paul. Both studies used an EPS protocol that included two sites of stimulation [right ventricular apex (RVA) and outflow tract (RVOT)] with up to three extrastimuli, with the FINGER protocol using a minimal coupling interval of 200 ms, whereas the Japanese investigators limited the coupling interval of the extrastimulus only by ventricular refractoriness. This difference in the EPS protocol probably explains why the percentage of asymptomatic individuals with inducible VF was higher in the Japanese study than in FINGER (57 % vs. 37 %). The latter figure is very close to the 34 % inducibility rate reported by Brugada among their asymptomatic individuals.
In Finger, asymptomatic individuals with inducible VF did have more spontaneous arrhythmic events than non-inducible patients, but the event rate was very low for both groups (1.1 vs. 0.4 % event rate per year). Moreover, using multivariate analysis the EPS results were no longer (independent) predictors of outcome. Inducibility of VF was not a predictor of outcome among asymptomatic individuals in the Japanese study.
Makimoto et al. [84] recently reported that inducibility to VF was of prognostic value only when VF was induced with one or two extrastimuli and suggested that triple extrastimulation should be avoided to prevent false-positive results. These results, however, where not confirmed by the prospective PRELUDE study [53]; the induction of VF was of no prognostic value regardless of the protocol of extrastimulation used.
Genetic Basis
Brugada syndrome shows an autosomal dominant mode of inheritance. The first gene to be linked to the syndrome is SCN5A, the gene that encodes the α subunit of the cardiac sodium channel gene [85]. Figure 29.1 highlights the diversity of SCN5A mutations associated with the Brugada syndrome. Of note, mutations in SCN5A are also responsible for the LQT3 form of the long QT syndrome and cardiac conduction disease. A number of mutations have been reported to cause overlapping syndromes; in some cases all three phenotypes are present [87]. Over 300 mutations in SCN5A have been associated with the syndrome in recent years [88].
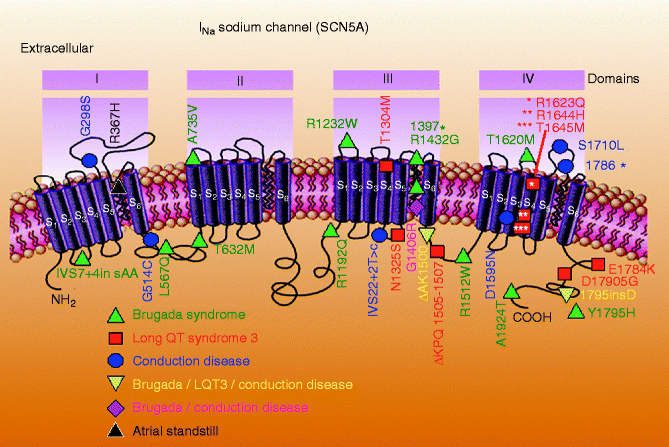
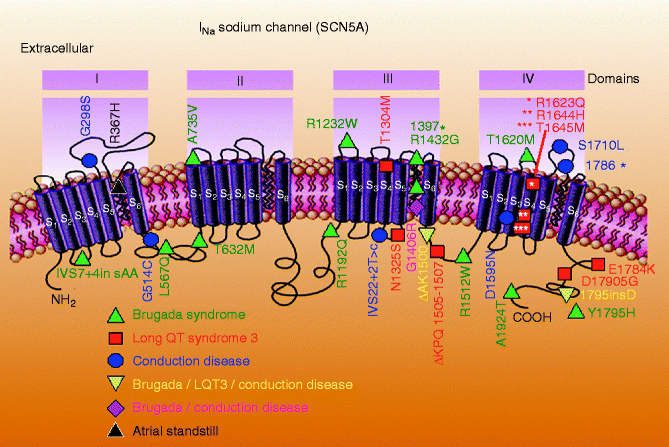
Figure 29–1.
Schematic of SCN5A, the gene that encodes the α subunit of the sodium channel, illustrating mutations linked to Brugada syndrome, long QT3 syndrome, conduction disease and atrial standstill. Some mutations are associated with combined phenotypes (From Antzelevitch and Viskin [86]. Reprinted with permission from Springer)
Only a fraction of these mutations have been studied in expression systems and shown to result in loss of function. A number of mechanisms have been delineated for the reduction in sodium channel current (INa), including [89–93]: (1) failure of the sodium channel to express due to faulty protein synthesis and/or trafficking; (2) a shift in the voltage- and time-dependence of sodium channel current (INa) activation, inactivation or reactivation; (3) entry of the sodium channel into an intermediate state of inactivation from which it recovers more slowly or (4) accelerated inactivation of the sodium channel. In in vitro expression systems, the premature inactivation of the sodium channel is sometimes observed at physiological temperatures, but not at room temperature [94]. Acceleration of INa inactivation was still more accentuated at higher than physiological temperatures, suggesting that the syndrome may be unmasked, and that patients with the Brugada syndrome may be at an increased risk, during a febrile state [94]. A number of Brugada patients displaying fever-induced polymorphic VT have been identified since the publication of this report [23, 61, 95–102].
Mutation in the SCN5A gene account for approximately 11–28 % of Brugada syndrome cases [88]. A higher incidence of SCN5A mutations has been reported in familial than in sporadic cases [90]. It is important to recognize that a negative SCN5A result does not rule out this gene as a cause, since the promoter region, cryptic splicing mutations or presence of gross rearrangements are generally not part of routine investigation. A recent report by Hong et al. [103] provided the first report of a dysfunctional sodium channel created by an intronic mutation giving rise to cryptic splice site activation in SCN5A in a family with the Brugada syndrome. The deletion of fragments of segments two and three of Domain IV of SCN5A caused complete loss of function.
Evidence in support of the hypothesis that an SCN5A promoter polymorphism common in Asians modulates variability in cardiac conduction, and may contribute to the high prevalence of Brugada syndrome in the Asian population was recently advance by Bezzina and co-workers [104]. Sequencing of the SCN5A promoter identified a haplotype variant consisting of six polymorphisms in near-complete linkage disequilibrium that occurred at an allele frequency of 22 % in Asian subjects and was absent in whites and blacks. These findings demonstrate that sodium channel transcription in the human heart may vary considerably among individuals and races and be associated with variable conduction velocity and arrhythmia susceptibility [104].
BrS has in recent years been linked to mutations in ten other genes. Calcium channel genes mutations, including those in CACNA1C (Cav1.2, BrS3), CACNB2b (Cavß2b, BrS4) and CACNA2D1 (Cavα2δ1, BrS9) are found in approximately 12–13 % of probands [105, 106]. Mutations in glycerol-3-phosphate dehydrogenase 1-like enzyme gene (GPD1L, BrS2), SCN1B (β1-subunit of Na channel, BrS5), KCNE3 (MiRP2; BrS6), SCN3B (β3-subunit of Na channel, BrS7), KCNJ8 (BrS8) and KCND3 (BrS10) are more rare [107–112]. Mutations in these genes lead to loss of function in INa and ICa, as well as to a gain of function in Ito or IK-ATP. MOG1 was recently described as a new partner of Nav1.5, playing a role in its regulation, expression and trafficking. A missense mutation in MOG1 has also been associated with BrS (BrS11) [113, 114].
It is generally accepted that identification of specific mutations may not be very helpful in formulating a diagnosis or providing a prognosis. Mutations have been reported throughout the SCN5A gene and no hotspots have been identified. It is not clear whether some mutations are associated with a greater risk of arrhythmic events or sudden death. Genetic testing is recommended for support of the clinical diagnosis, for early detection of relatives at potential risk and particularly for the purpose of advancing research and our understanding of genotype-phenotype relations [115].
Cellular and Ionic Mechanisms Underlying the Development of the Brugada Phenotype
Transmural Cellular and Ion Channel Distinctions
Ventricular myocardium is known to be comprised of at least three electrophysiologically and functionally distinct cell types: epicardial, M and endocardial cells [116, 117]. These three principal ventricular myocardial cell types differ with respect to phase 1 and phase 3 repolarization characteristics. Ventricular epicardial and M, but not endocardial, cells generally display a prominent phase 1, due to a large 4-aminopyridine (4-AP) sensitive transient outward current (Ito), giving the action potential a spike and dome or notched configuration. These regional differences in Ito, first suggested on the basis of action potential data [118], have now been directly demonstrated in canine [119], feline [120], rabbit [121], rat [122], and human [123–126] ventricular tissues and cells. Differences in the magnitude of the action potential notch and corresponding differences in Ito have also been described between right and left ventricular epicardium [127]. Similar inter-ventricular differences in Ito have also been described for canine ventricular M cells [128]. This distinction is thought to form the basis for why the Brugada syndrome, a channelopathy–mediated form of sudden death, is a right ventricular disease.
The molecular basis for the transmural distribution of Ito has long been a subject of debate. The transmural gradient of Ito in dog has been ascribed to a transmural distribution of: (1) KCND3 gene (Kv4.3), which encodes the α subunit of the Ito channel [129]; (2) KChIP2, a β subunit that co-assembles with Kv4.3 [130] and (3) IRX5, a transcriptional factor regulating KCND3 [131].
Myocytes isolated from the epicardial region of the left ventricular wall of the rabbit show a higher density of cAMP-activated chloride current when compared to endocardial myocytes [132]. Ito2, initially ascribed to a K+ current, is now thought to be primarily due to the calcium-activated chloride current (ICl(Ca)) is also thought to contribute to the action potential notch, but it is not known whether this current, differs among the three ventricular myocardial cell types [133].
Recent studies involving canine ventricular myocytes have shown that calcium current (ICa) is similar among cells isolated from epicardium, M, and endocardial regions of the left ventricular wall [134, 135]. One study however reported differences in Ca2+ channel properties between epicardial and endocardial canine ventricular cells. In that study, ICa was found to be larger in endocardial than in epicardial myocytes (3.4 ± 0.2 vs. 2.3 ±0.1 pA/pF). A low-threshold, rapidly activating and inactivating Ca2+ current that resembles the T-type current, was also recorded in all endocardial myocytes, but was small or absent in epicardial myocytes. The T-like current was comprised of two components: a Ni2+-sensitive T-type current and a tetrodotoxin-sensitive Ca2+ current [136].
The surface epicardial and endocardial layers are separated by transitional and M cells. M cells are distinguished by the ability of their action potential to prolong disproportionately relative to the action potential of other ventricular myocardial cells in response to a slowing of rate and/or in response to action potential duration (APD)-prolonging agents [116, 137, 138]. In the dog, the ionic basis for these features of the M cell include the presence of a smaller slowly activating delayed rectifier current (IKs) [139], a larger late sodium current (late INa) [140] and a larger Na-Ca exchange current (INa-Ca) [141]. In the canine heart, the rapidly activating delayed rectifier (IKr) and inward rectifier (IK1) currents are similar in the three transmural cell types. Transmural and apico-basal differences in the density of IKr channels have been described in the ferret heart [142]. IKr message and channel protein are much larger in the ferret epicardium. IKs is larger in M cells isolated from the right vs. left ventricles of the dog [128].
Cellular Basis for the Electrocardiographic J Wave
The presence of a prominent action potential notch in epicardium but not endocardium gives rise to a transmural voltage gradient during ventricular activation that manifests as a late delta wave following the QRS or what more commonly is referred to as a J wave [4] or Osborn wave. A distinct J wave is often observed under baseline conditions in the ECG of some animal species, including dogs and baboons. Humans more commonly display a J point elevation rather than a distinct J wave. A prominent J wave in the human ECG is considered pathognomonic of hypothermia [143–145] or hypercalcemia [146, 147].
A transmural gradient in the distribution of Ito is responsible for the transmural gradient in the magnitude of phase 1 and action potential notch, which in turn gives rise to a voltage gradient across the ventricular wall responsible for the inscription of the J wave or J point elevation in the ECG [118, 119, 148]. Direct evidence in support of the hypothesis that the J wave is caused by a transmural gradient in the magnitude of the Ito-mediated action potential notch derives from experiments conducted in the arterially-perfused right ventricular wedge preparation showing a correlation between the amplitude of the epicardial action potential notch and that of the J wave recorded during interventions that alter the appearance of the electrocardiographic J wave, including hypothermia, premature stimulation (restitution), and block of Ito by 4-aminopyridine (4-AP) (Fig. 29.2) [4].
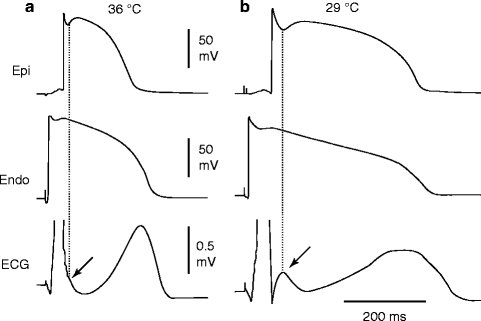
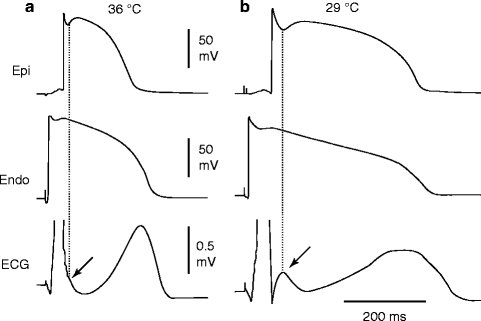
Figure 29–2.
Hypothermia-induced J wave. Each panel shows transmembrane action potentials from the epicardial and endocardial regions of an arterially perfused canine left ventricular wedge and a transmural ECG simultaneously recorded. (a) The relatively small action potential notch in epicardium but not in endocardium is associated with an elevated J-point at the R-ST junction (arrow) at 36 °C. (b) A decrease in the temperature of the perfusate to 29 °C results in an increase in the amplitude and width of the action potential notch in epicardium but not endocardium, leading to the development of a transmural voltage gradient that manifests as a prominent J wave on the ECG (arrow) (Modified from Yan and Antzelevitch [4], with permission from Wolters Kluwer Health)
Transmural activation within the thin wall of right ventricle (RV) is relatively rapid causing the J wave to be buried inside the QRS. Thus, although the action potential notch is most prominent in right ventricular epicardium, right ventricular myocardium would be expected to contribute relatively little to the manifestation of the J wave under normal conditions. These observations are consistent with the manifestation of the normal J wave in ECG leads in which the mean vector axis is transmurally oriented across the left ventricle and septum. Accordingly, the J wave in the dog is most prominent in leads II, III, aVR, aVF, and mid to left precordial leads V3 through V6. A similar picture is seen in the human ECG [147, 149]. In addition, vectorcardiography indicates that the J wave forms an extra loop that occurs at the junction of the QRS and T loops [150]. It is directed leftward and anteriorly, which explains its prominence in leads associated with the left ventricle.
The first description of the J wave was in the 1920s in animal experiments involving hypercalcemia [146]. The first extensive description and characterization appeared 30 years later by Osborn in a study involving experimental hypothermia in dogs [151]. The appearance of a prominent J wave in the clinic is typically associated with pathophysiological conditions, including hypothermia [143, 149] and hypercalcemia [146, 147]. The prominent J wave induced by hypothermia is the result of a marked accentuation of the spike-and-dome morphology of the action potential of M and epicardial cells (i.e., an increase in both width and magnitude of the notch). In addition to inducing a more prominent notch, hypothermia produces a slowing of conduction which permits the epicardial notch to clear the QRS so as to manifest a distinct J wave. Hypercalcemia-induced accentuation of the J wave [146, 147, 152] may also be explained on the basis of an accentuation of the epicardial action potential notch, possibly as a result of an augmentation of the calcium-activated chloride current and a decrease in ICa [153]. Accentuation of the action potential notch also underlies the electrocardiographic and arrhythmogenic manifestations of the Brugada syndrome.
Repolarization Abnormalities as the Basis for the ECG and Arrhythmic Manifestations of BrS. Exaggeration of the J Wave Is the Basis for ST Segment Elevation in BrS
An increase in net repolarizing current, whether secondary to a decrease of inward currents or an increase of outward current, accentuates the action potential notch leading to augmentation of the J wave or appearance of ST segment elevation. A further increase in net repolarizing current can result in partial or complete loss of the action potential dome, leading to a transmural voltage gradient that manifests as accentuation of the J wave or an ST segment elevation [154–156]. In regions of the myocardium exhibiting a prominent Ito, such as the right ventricular epicardium, marked accentuation of the action potential notch leads to a augmentation of the J wave, manifesting as a coved-type ST segment elevation, which is diagnostic of BrS (Fig. 29.3a, b). Because the second action potential upstroke is delayed, epicardium now repolarizes after endocardium, causing inversion of the T wave. Note that although the ECG now displays the typical BrS morphology with a covered type ST segment elevation and negative T wave, no major arrhythmogenic substrates are present. The substrate for reentry, however develops with any additional outward shift of the net current active during the early phase of the action potential. This leads to loss of the action potential dome, thus creating a dispersion of repolarization between epicardium and endocardium as well as within epicardium, between the region where the dome is lost and regions at which it is maintained (Fig. 29.3c). Sodium channel blockers like procainamide, pilsicainide, propafenone, flecainide and disopyramide cause a further outward shift of current flowing during the early phases of the action potential and are thereby effective in inducing or unmasking ST segment elevation in patients with concealed J-wave syndromes [22, 31, 158]. Sodium channel blockers like quinidine, which also inhibits Ito, reduce the magnitude of the J wave and normalize ST segment elevation [154, 159]. Loss of the action potential dome is usually heterogeneous, resulting in marked abbreviation of action potential at some sites but not others. The dome can then propagate from regions at which it is maintained to regions where it is lost, giving rise to a very closely coupled extrasystole via phase 2 reentry (Fig. 29.3d) [3]. The phase 2 reentrant beat is capable of initiating polymorphic ventricular tachycardias (VT) or ventricular fibrillation (Fig. 29.3e, f).
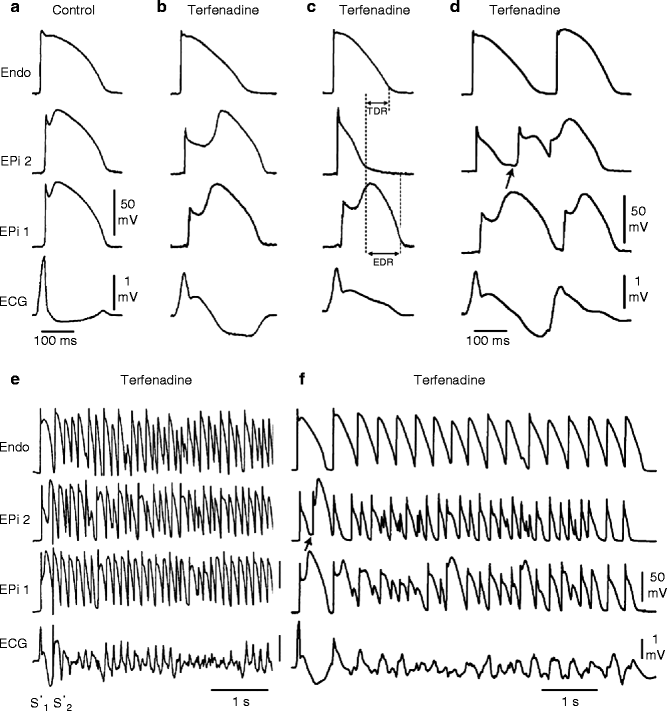
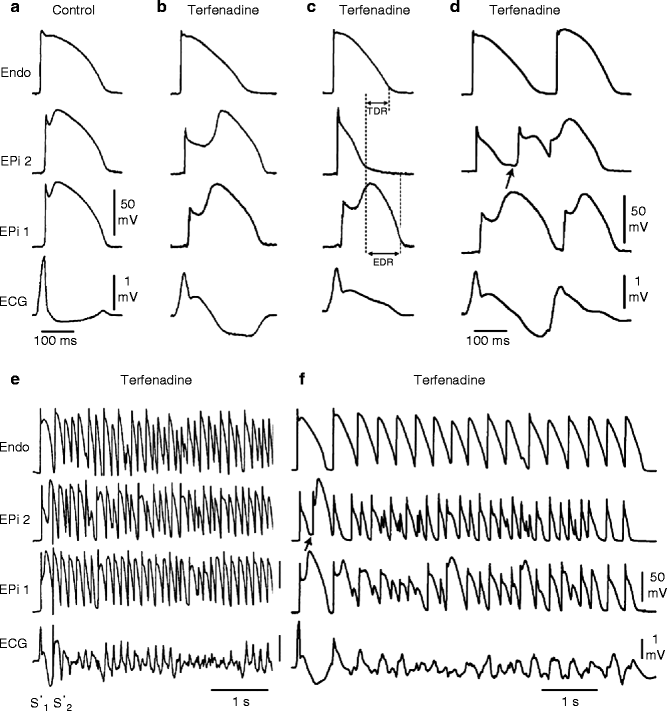
Figure 29–3.
Cellular basis for electrocardiographic and arrhythmic manifestation of BrS. Each panel shows transmembrane action potentials from one endocardial (top) and two epicardial sites together with a transmural ECG recorded from a canine coronary-perfused right ventricular wedge preparation. (a) Control (Basic cycle length (BCL) 400 ms). (b) Combined sodium and calcium channel block with terfenadine (5 μM) accentuates the epicardial action potential notch creating a transmural voltage gradient that manifests as an exaggerated J wave or ST segment elevation in the ECG. (c) Continued exposure to terfenadine results in all-or-none repolarization at the end of phase 1 at some epicardial sites but not others, creating a local epicardial dispersion of repolarization (EDR) as well as a transmural dispersion of repolarization (TDR). (d) Phase 2 reentry occurs when the epicardial action potential dome propagates from a site where it is maintained to regions where it has been lost giving rise to a closely coupled extrasystole. (e) Extrastimulus (S1–S2 = 250 ms) applied to epicardium triggers a polymorphic VT. (f) Phase 2 reentrant extrasystole triggers a brief episode of polymorphic VT (Modified from Fish and Antzelevitch [157], with permission from Elsevier Limited)
Phase 2 reentry has been shown to occur when right ventricular epicardium is exposed to: (1) K+ channel openers such as pinacidil [160]; (2) sodium channel blockers such as flecainide [3]; (3) increased [Ca2+]o [153]; (4) calcium channel blockers such as verapamil; (5) metabolic inhibition [161]; and (6) simulated ischemia [162]. Evidence in support of a phase 2 reentrant mechanism in humans was provided by Thomsen et al. [163, 164].
Does Delayed Conduction Contribute to the ECG and Arrhythmic Manifestations of BrS?
While most investigations suggest that repolarization abnormalities underlie the pathophysiology of Brugada syndrome, recent studies suggest the possibility of delayed depolarization in the right ventricular outflow tract as a contributing mechanism to ST segment elevation or J waves associated with BrS [165, 166]. The repolarization vs. depolarization hypotheses controversy has been documented as a published debate [167].
Seemingly compelling data in support of delayed conduction in the RVOT as the basis for BrS was recently presented by Nademanee and co-workers [166]. Using an electrogram applied to the epicardial surface of the RVOT, these authors recorded late potentials, in some cases appearing as a continuous fractionated electrogram. This was interpreted as indicating delayed conduction over the anterior aspect of the RVOT epicardium. Catheter ablation over this abnormal region of the RVOT resulted in normalization of the Brugada ECG pattern over a period of up to 3 months and prevented VT/VF, both during electrophysiological studies, as well as spontaneous recurrence of VT/VF episodes. Signal averaged ECG (SAECG) recordings have also demonstrated late potentials in patients with the Brugada syndrome, especially in the anterior wall of the right ventricular outflow tract (RVOT) [168–174].
Although traditionally ascribed to conduction delays secondary to structural defects, in cases of BrS, late potentials of the type described by Nademanee et al. more likely arise from a delayed second upstroke of the epicardial action potential in the RVOT or to a concealed phase 2 reentry [19, 167, 170]. It is noteworthy that wall motion abnormalities have also been detected in BrS patients [175]. Although such contractile abnormalities are commonly considered pathognomonic of structural disease, in the setting of BrS, they could well be the result of loss of the action potential dome in the right ventricular epicardium [170, 176]. Loss of the dome would lead to contractile dysfunction because calcium entry into the cells would be greatly diminished and sarcoplasmic reticulum calcium stores would be depleted.
It has been suggested that the rate-dependence of the ST segment elevation in BrS may be helpful in discriminating between the repolarization and depolarization hypotheses [19]. If the ST segment elevation is due predominantly to delayed conduction in the RVOT, acceleration of the rate would be expected to further aggravate conduction and thus accentuate the ST segment elevation and the RBBB morphology of the ECG. If, on the other hand, ST segment elevation or the BrS sign is secondary to accentuation of the epicardial action potential notch, acceleration of the rate would be expected to normalize the ECG, by reducing the action potential notch and restoring the action potential dome in RV epicardium. This occurs because the transient outward current, which is at the heart of this mechanism, is slow to recover from inactivation and is less available at faster rates.
Although there are relatively few reports of the effects of pacing, most investigators in the field agree that there is a tendency for the ST segment elevation associated with Brugada ECGs to normalize during an increase in heart rate, consistent with the repolarization hypothesis [177–179]. There are however also reports of ST segment elevation or J point elevation with exercise. Amin et al. recently reported J point elevation in BrS patients (both SCN5A+ and SCN5A−) during exercise [180]. The principal difference between studies showing a decrease vs. increase of the J point in response to exercise appears to be the presence of a prominent ST segment elevation at baseline in the former.
Interestingly, in BrS cases in which ST segment elevation is accompanied by notching of the QRS, the latter suggesting major conduction delay in the RVOT, exercise-induced acceleration of rate leads to further fragmentation of the QRS, but to a normalization of the ST segment elevation in all right precordial leads [167]. These findings suggest that the ST segment elevation is due to a repolarization defect and not to the clearly apparent depolarization defect responsible for the fragmentation of the QRS. Fragmentation of the QRS is associated with increased mortality and arrhythmic events in patients with coronary artery disease as well as patients with arrhythmogenic right ventricular cardiomyopathy and Brugada syndrome [181].
Kurita et al. placed monophasic action potential (MAP) electrodes on the epicardial and endocardial surfaces of the right ventricular outflow tract (RVOT) in patients with the Brugada syndrome and demonstrated an accentuated notch in the epicardial response, thus providing direct support for the repolarization hypothesis in humans [182, 183].
Additional support for the repolarization hypothesis derives from the demonstration that quinidine normalizes ST segment elevation and suppresses late potentials recorded in patients with Brugada syndrome [184–186]. These effects of the drug are presumably due to inhibition of Ito leading to reduction of the epicardial action potential notch and normalization of the repolarization heterogeneities. If the ST segment elevation or the late potentials were due to delayed conduction, quinidine-induced INa inhibition would be expected to accentuate their appearance.
Although there is no experimental model of BrS based on the depolarization hypothesis, several groups have developed a models of BrS based on the repolarization hypothesis [154, 157, 187–192].
Experimental models displaying major conduction delays have been developed by exposing wedge preparations to global ischemia [193, 194]. Progressive delay in these models leads to a gradual prolongation of the R wave and inversion of the T wave. The ECG at first glance resembles a BrS phenotype, but with closer inspection it is clear that the depolarization hypothesis does not lead to an ST segment elevation, but rather to an R wave prolongation. The marked conduction delay is due to a discontinuity in conduction in the deep subepicardium. Conduction under these conditions is exquisitely sensitive to rate, with acceleration leading to block and inexcitability. This is a common characteristic of ischemia-induced tombstone morphology of the ECG induced by coronary spasm [195], but is not characteristic of BrS A similar phenomenon is observed in right ventricular wedge preparations following exposure to hyperkalemic conditions [196].
Of note, magnetocardiograms recorded from patients with complete RBBB have been shown to generate currents from the RVOT to the upper left chest that are opposite from those recorded in patients with Brugada syndrome [197].
While the available data, both basic and clinical, point to the repolarization hypothesis, i.e., transmural voltage gradients that develop secondary to accentuation of the epicardial notch, at times leading to loss of the action potential dome, as the predominant mechanism underlying the Brugada syndrome ECG signature and arrhythmogenesis, there is no doubt that in some cases, particularly those associated with sodium channel loss of function, conduction slowing may contribute to the development of arrhythmias. There are also likely to be cases in which a conduction defect may predominate [198, 199].
Parasympathetic agonists like acetylcholine facilitate loss of the action potential dome [200] by suppressing ICa and/or augmenting potassium current. β(beta)-adrenergic agonists restore the dome by augmenting ICa. Sodium channel blockers also facilitate loss of the canine right ventricular action potential dome via a negative shift in the voltage at which phase 1 begins [2, 3]. These findings are consistent with accentuation of ST segment elevation in patients with the Brugada syndrome following vagal maneuvers or Class I antiarrhythmic agents as well as normalization of the ST segment elevation following β(beta) adrenergic agents and phosphodiesterase III inhibitors [4, 5, 201]. Loss of the action potential dome is more readily induced in right vs. left canine ventricular epicardium [127, 161, 202] because of the more prominent Ito-mediated phase 1 in action potentials in this region of the heart. This distinction is believed to be the basis for why the Brugada syndrome is a right ventricular disease.
T Wave Alternans
T wave alternans (TWA) is characterized by beat to beat alteration in the amplitude, polarity and/or morphology of the electrocardiographic T wave. TWA has been reported in patients with the Brugada syndrome (BS) and is thought to be associated with an increased risk for development of VT/VF [158, 203–209]. Experimental studies suggest that T wave alternans is due to at least two cellular mechanism, including: (1) loss of the epicardial action potential dome on alternate beats (Fig. 29.4) and (2) concealed phase 2 reentry on alternating beats (Fig. 29.5) [157, 210–212]. T wave alternans developing after administration of sodium channel blockers has also been associated with a high risk of clinical VT/VF in patients with BrS [213].
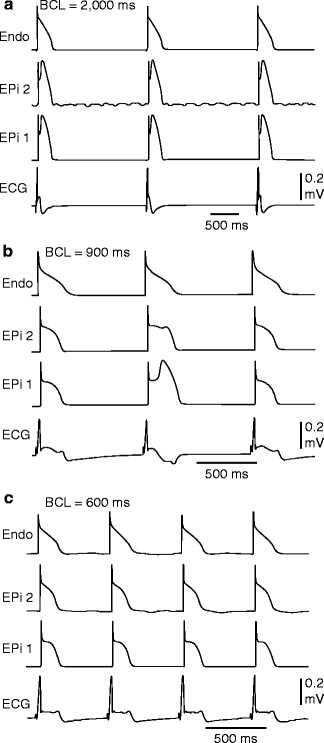
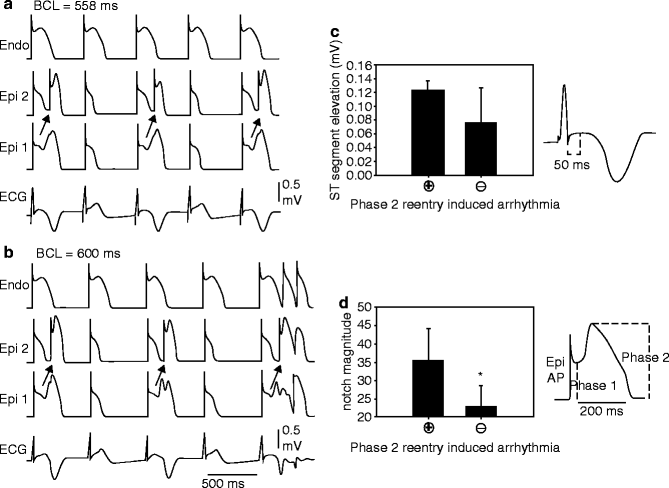
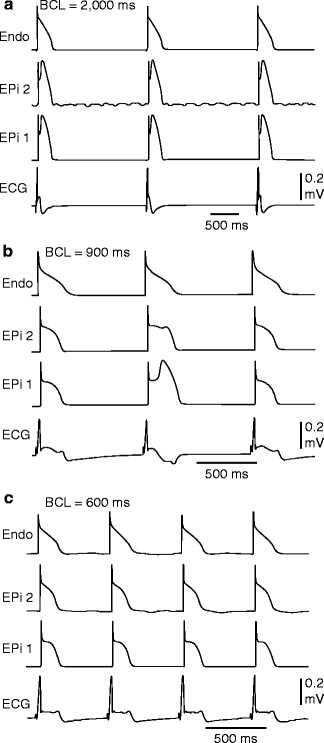
Figure 29–4.
Verapamil (1 μM)-induced loss of the epicardial action potential dome in alternate beats causes T wave alternans in a canine arterially-perfused right ventricular wedge preparation. (a) At a BCL of 2,000 ms, endocardial and epicardial action potentials repolarize almost simultaneously, generating little or no T wave on the ECG. (b) Decreasing the cycle length to 900 ms induces heterogeneous loss of the epicardial action potential dome in alternate beats while the endocardial response remains constant, resulting in profound T wave alternans. (c) Decreasing the cycle length to 600 ms leads to homogeneous loss of the action potential dome on all beats, leading to ST segment elevation but no T wave alternans in the ECG (Modified from Fish and Antzelevitch [210], with permission from John Wiley and Sons)
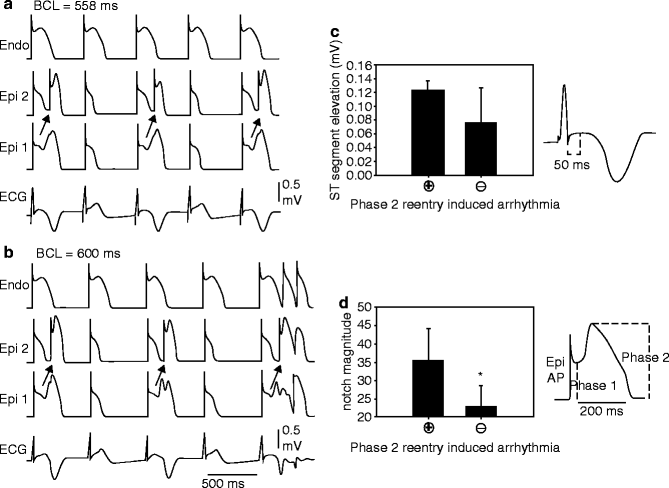
Figure 29–5.
Verapamil (1 μM)-induced concealed phase 2 reentry in alternate beats leading to T wave alternans in the coronary-perfused right ventricular wedge preparation. (a) T wave alternans occurs as a result of concealed phase 2 reentry. The dome propagates from Epi 1 to Epi 2 on alternating beats while the endocardial response remains constant. The concealed phase 2 reentry results in a negative T wave. BCL = 558 ms. (b) Increasing the cycle length to 600 ms exaggerates the T wave alternans. The phase relationship between Epi 1, Epi 2, and Endo shifts slightly, allowing the previously concealed phase 2 reentry to propagate transmurally, leading to two extrasystoles. (c) The ST segment elevation, measured 50 ms after the end of the QRS, is greater during alternans secondary to concealed phase 2 reentry compared to alternans due to alternating loss of the epicardial action potential dome (n = 10 phase 2 reentry + and 12 phase 2 reentry – episodes, *p = 0.027). (d) The size of the epicardial action potential notch magnitude (ph2 amp – ph1 amp/ph 2 amp) at BCL 2,000 ms during control was significantly smaller in preparations not displaying phase 2 reentry-induced arrhythmias versus those that did (n = 5 for each category, *p = 0.025) (Modified from Fish and Antzelevitch [210], with permission from John Wiley and Sons)
Acquired Forms of Brugada Syndrome and Modulating Factors
The electrocardiographic and arrhythmic manifestations of the Brugada syndrome can be induced and modulated by a large number of agents and factors. The Brugada ECG can be unmasked or modulated by sodium channel blockers, a febrile state, vagotonic agents, α adrenergic agonists, β adrenergic blockers, tricyclic or tetracyclic antidepressants, first generation antihistaminics (dimenhydrinate), a combination of glucose and insulin, hyperkalemia, hypokalemia, hypercalcemia, and by alcohol and cocaine toxicity (Fig. 29.6) [5, 21, 22, 214–221]. These agents may also induce acquired forms of the Brugada syndrome (Table 29.2). An up-to-date list of agents to avoid in Brugada syndrome can be found at www.BrugadaDrugs.org.
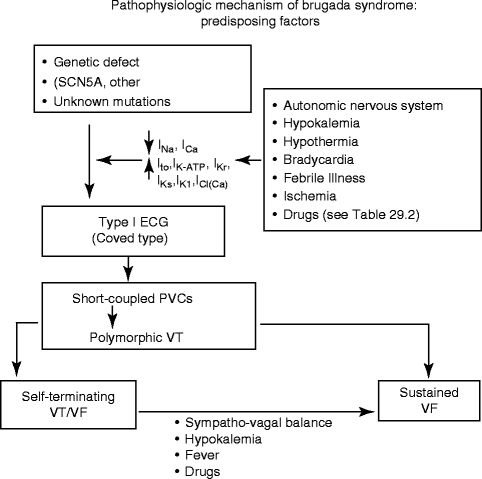
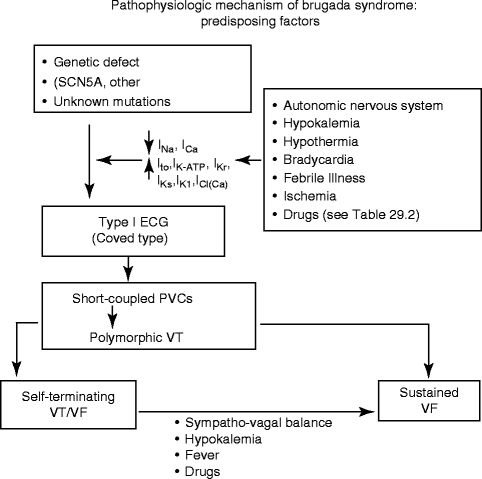
Figure 29–6.
Factors predisposing to the electrocardiographic and arrhythmic manifestations of the Brugada syndrome (From Antzelevitch and Viskin [86]. Reprinted with permission from Springer)
Table 29–2.
Drug-induced Brugada-like ECG patterns
I. Antiarrhythmic drugs |
1. Na+ channel blockers |
2. Ca2+ channel blockers |
3. β Blockers |
Propranolol intoxication [232] |
II. Antianginal drugs |
1. Ca2+ channel blockers |
Nifedipine, diltiazem |
2. Nitrate |
Isosorbide dinitrate, nitroglycerine [233] |
3. K+ channel openers |
Nicorandil |
III. Psychotropic drugs |
Maprotiline [238] |
2. Phenothiazine |
3. Selective serotonin reuptake inhibitors |
Fluoxetine [239] |
IV. Other drugs |
1. Histaminic H1 receptor antagonists |
Dimenhydrinate [217] |
Diphenhydramine [247] |
3. Alcohol intoxication |
6. Ergonovine [253] |
Myocardial infarction or acute ischemia due to vasospasm involving the RVOT mimics ST-segment elevation similar to that seen in Brugada syndrome. This effect is secondary to the depression of ICa, inactivation of INa and the activation of IK-ATP during ischemia, and suggests that patients with congenital and possibly acquired forms of Brugada syndrome may be at a higher risk for ischemia-related sudden cardiac death [253, 255]. Although the coexistence of Brugada syndrome and vasospastic angina in the same patient is not rare, Chinushi et al. have failed to observe an enhanced susceptibility to VF nor a proarrhythmic effect of Ca-antagonist in this setting [256].
Hypokalemia has been suggested to be a contributing factor in the high prevalence of Sudden unexpected nocturnal death syndrome (SUDS) in the Northeastern region of Thailand where potassium deficiency is endemic [220, 257]. Serum potassium in the Northeastern population is significantly lower than that of the population in Bangkok, which lies in the central part of Thailand where potassium is abundant in the food. Hypokalemia has been reported to induce VF in a 60 year old man who had asymptomatic Brugada syndrome, without a family history of sudden cardiac death [220]. This patient was initially treated for asthma by steroids, which lowered serum potassium from 3.8 mM on admission to 3.4 and 2.9 mM on the 7th day and 8th day of admission, respectively. Both were associated with unconsciousness. VF was documented during the last episode, which reverted spontaneously to sinus rhythm. Hypokalemia may exert these effects by increasing the chemical gradient for K+ and thus the intensity of Ito.
VT/VF and sudden death in the Brugada syndrome usually occur at rest and at night. Circadian variation of sympatho-vagal balance, hormones and other metabolic factors are likely to contribute to this circadian pattern. Bradycardia, due to altered sympatho-vagal balance or other factors, may contribute to arrhythmia initiation [26, 54, 55]. Abnormal 123I-MIBG uptake in 8 (17 %) of the 17 Brugada syndrome patients but none in the control group was demonstrated by Wichter et al. [258]. Segmental reduction of 123I-MIBG in the inferior and the septal left ventricular wall was observed indicating presynaptic sympathetic dysfunction. Of note, imaging of the right ventricle, particularly the RVOT, is difficult with this technique, so that insufficient information is available concerning sympathetic function in the regions known to harbor the arrhythmogenic substrate. Moreover, it remains unclear what role the reduced uptake function plays in the arrhythmogenesis of the Brugada syndrome. If the RVOT is similarly affected, this defect may indeed alter the sympatho-vagal balance in favor of the development of an arrhythmogenic substrate [154, 200].
The Thai Ministry of Public Health Report (1990) found an association between a large meal on the night of death in Sudden unexplained nocturnal death syndrome (SUNDS) patients [257]. Consistent with this observation, a recent study by Nogami et al. found that glucose and insulin could unmask the Brugada ECG [219]. Another possibility is that sudden death in these patients is due to the increased vagal tone produced by the stomach distention. A recent study by Ikeda et al. [24] has shown that a full stomach after a large meal can unmask a Type I ECG, particularly in Brugada syndrome patients at high risks for arrhythmic events, thus suggesting that this technique may be of diagnostic and prognostic value.
Accelerated inactivation of the sodium channel caused by SCN5A mutations associated with the Brugada syndrome has been shown to be accentuated at higher temperatures [94] suggesting that a febrile state may unmask the Brugada syndrome. Indeed, several case reports have emerged recently demonstrating that febrile illness could reveal the Brugada ECG and precipitate VF [23, 95, 96, 259–261]. A report from Keller et al. [262] has identified a missense mutation, F1344S, in SCN5A in a patient with Brugada syndrome and fever-induced VT/VF. Expression of F1344S showed a shift in the voltage-dependence of activation, which was further accentuated at high temperatures mimicking fever. Thus fever may also cause a loss of function in INa by accelerating inactivation as well as producing a shift in the voltage-dependence of activation.
Anecdotal data point to hot baths as a possible precipitating factor. Of note, the Northeastern part of Thailand, where the Brugada syndrome is most prevalent, is known for its very hot climate. A study is underway to assess whether this extreme climate influences the prognosis of the disease.
Sex-Related Differences in the Manifestation of the Brugada Syndrome
Although the mutation responsible for the Brugada syndrome is equally distributed between the sexes, the clinical phenotype is eight to ten times more prevalent in males than in females. The basis for this sex-related distinction has been shown to be due to a more prominent Ito-mediated action potential notch in the right ventricular (RV) epicardium of males vs. females (Figs. 29.7 and 29.8) [263]. The more prominent Ito causes the end of phase 1 of the RV epicardial action potential to repolarize to more negative potentials in tissues and arterially perfused wedge preparations from males, facilitating loss of the action potential dome and the development of phase 2 reentry and polymorphic VT.
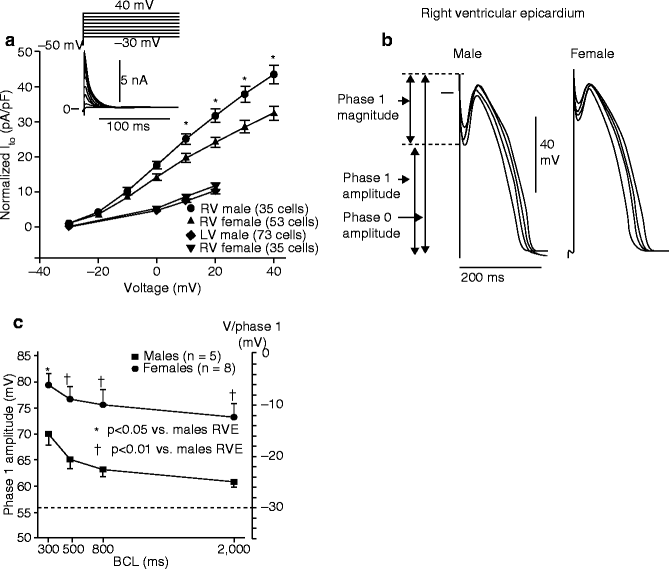
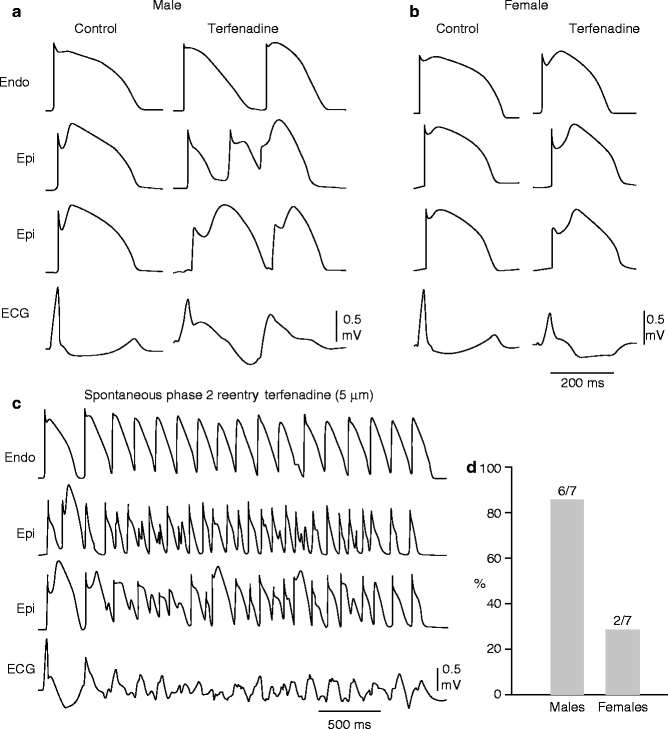
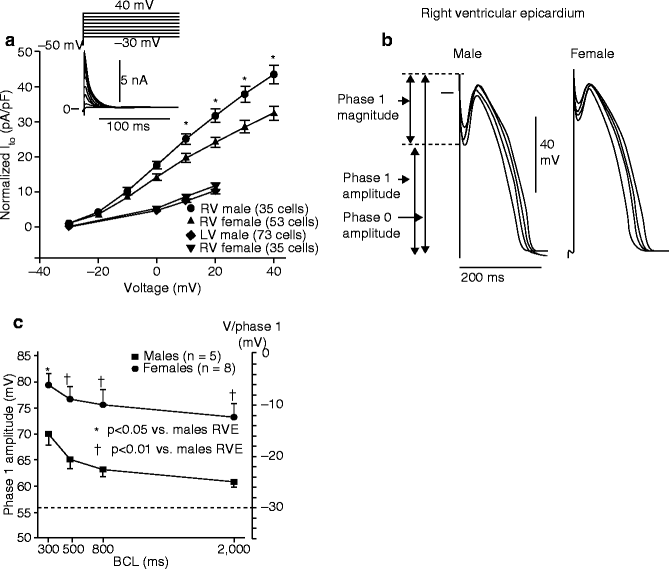
Figure 29–7.
Sex-based and inter-ventricular differences in Ito. (a) Mean I-V relationship for Ito recorded from RV epicardial cells isolated from hearts of male and female dogs. Inset: Representative Ito current traces and voltage protocol. Ito density was significantly greater in male vs female RV epicardial cells. No sex differences were observed in LV. (b) Transmembrane action potentials recorded from isolated canine RV epicardial male and female tissue slices. BCLs 300, 500, 800, and 2,000 ms. (c) Rate dependence of phase 1 amplitude and voltage at end of phase 1 (V/phase 1, mV) in males (solid squares) vs females (solid circles) (Modified from Di Diego et al. [263] with permission from Wolters Kluwer Health)
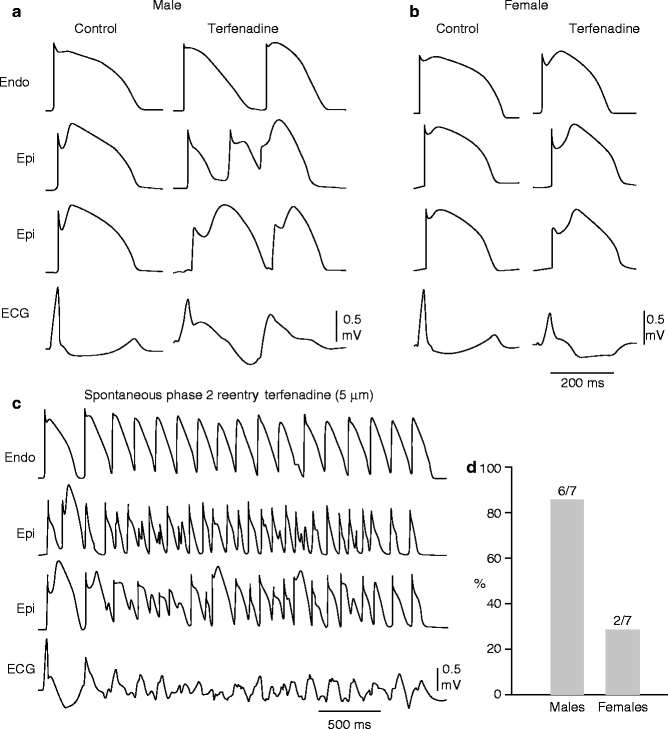
Figure 29–8.
Terfenadine induces Brugada phenotype more readily in male than female RV wedge preparations. Each panel shows action potentials recorded from two epicardial sites and one endocardial site, together with a transmural ECG. Control recordings were obtained at a BCL of 2,000 ms, whereas terfenadine data were recorded at a BCL of 800 ms after a brief period of pacing at a BCL of 400 ms. (a) Terfenadine (5 uM)-induced, heterogeneous loss of action potential dome, ST-segment elevation, and phase 2 reentry (arrow) in a male RV wedge preparation. (b) Terfenadine fails to induce Brugada phenotype in a female RV wedge preparation. (c) Polymorphic VT triggered by spontaneous phase 2 reentry in a male preparation. (d) Incidence of phase 2 reentry in male (six of seven) vs female (two of seven) RV wedge preparations when perfused with 5 uM terfenadine for up to 2 h (Modified from Di Diego et al. [263], with permission from Wolters Kluwer Health)
Clinical and basic studies suggest that testosterone plays an important role in ventricular repolarization. Ezaki and co-workers [264] recently demonstrated that ST segment elevation is relatively small and similar in males and females before puberty. After puberty, ST segment elevation in males, but not females, increased dramatically, more so in the right precordial leads and decreased gradually with advancing age. The effect of androgen-deprivation therapy on the ST segment was also evaluated in 21 prostate cancer patients. Androgen-deprivation therapy significantly decreased ST segment elevation. These results suggest that testosterone modulates the early phase of ventricular repolarization and thus ST segment elevation.
The proposed cellular mechanism for the Brugada syndrome is summarized in Fig. 29.9. Available data support the hypothesis that the Brugada syndrome results from amplification of heterogeneities intrinsic to the early phases of the action potential among the different transmural cell types. This amplification is secondary to a rebalancing of currents active during phase 1, including a decrease in INa or ICa or augmentation of any one of a number of outward currents. ST segment elevation similar to that observed in patients with the Brugada syndrome occurs as a consequence of the accentuation of the action potential notch, eventually leading to loss of the action potential dome in right ventricular epicardium, where Ito is most prominent. Loss of the dome gives rise to both a transmural as well as epicardial dispersion of repolarization. The transmural dispersion is responsible for the development of ST segment elevation and the creation of a vulnerable window across the ventricular wall, whereas the epicardial dispersion give to phase 2 reentry which provides the extrasystole that captures the vulnerable window, thus precipitating VT/VF. The VT generated is usually polymorphic, resembling a very rapid form of Torsade de Pointes.
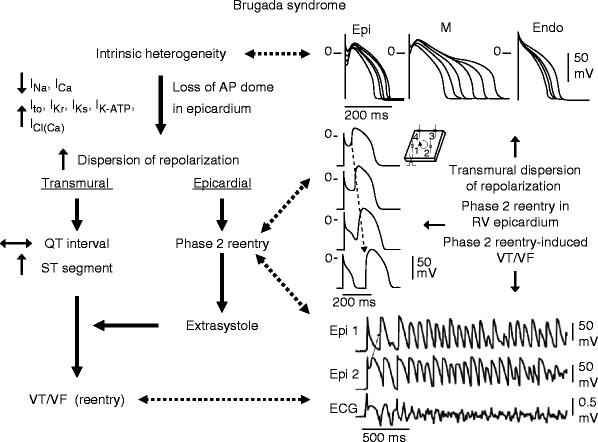
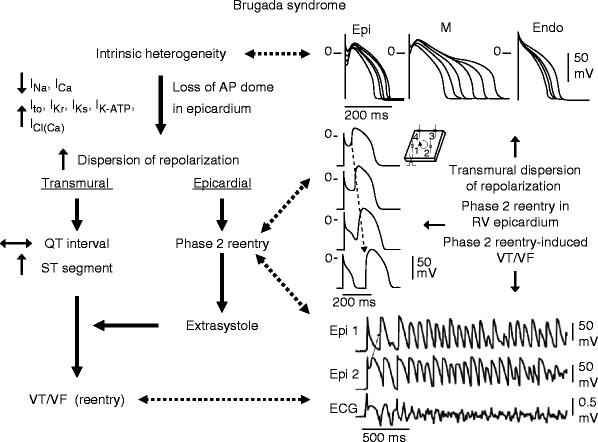
Figure 29–9.
Proposed mechanism for the Brugada syndrome. A shift in the balance of currents serves to amplify existing heterogeneities by causing loss of the action potential dome at some epicardial, but not endocardial sites. A vulnerable window develops as a result of the dispersion of repolarization and refractoriness within epicardium as well as across the wall. Epicardial dispersion leads to the development of phase 2 reentry, which provides the extrasystole that captures the vulnerable window and initiates VT/VF via a circus movement reentry mechanism (Modified from Antzelevitch [265], with permission from Oxford University Press)
Approach to Therapy
Device Therapy
The only proven effective therapy for the Brugada syndrome is an implantable cardioverter defibrillator (ICD) (Table 29.3) [266, 283]. Recommendations for ICD implantation from the Second Consensus Conference [9, 10] are presented in Fig. 29.10 and are summarized as follows:
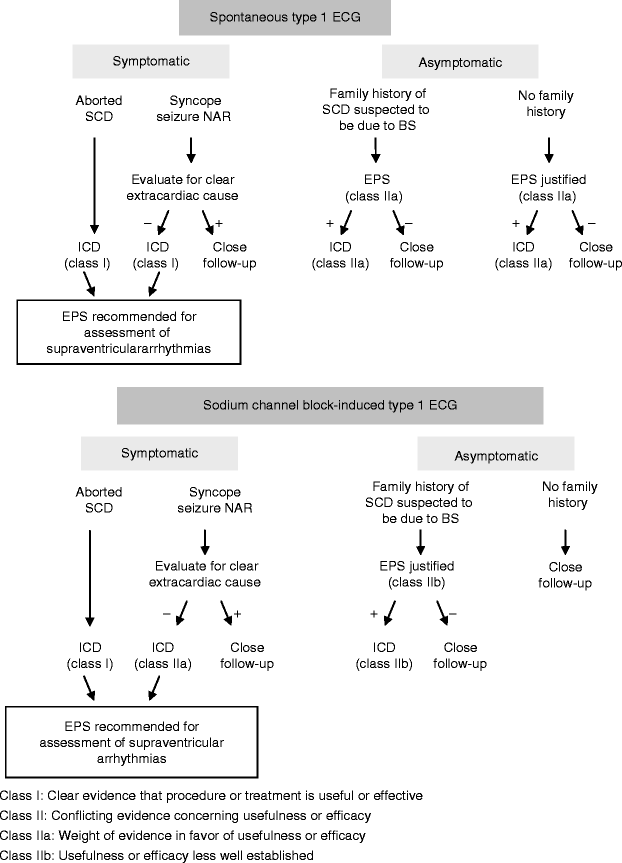
Table 29–3.
Device and pharmacologic approach to therapy
Devices and ablation |
ICD [266] |
? Pacemaker [268] |
Pharmacologic approach to therapy |
Ineffective |
Amiodarone [75] |
β Blockers [75] |
Class IC antiarrhythmics |
Flecainide [31] |
Propafenone [226] |
? Disopyramide [269] |
Class IA antiarrhythmics |
Procainamide [22] |
Effective for treatment of electrical storms |
Orciprenaline (Kyriazis et al. [271]) |
Phosphodiesterase III inhibitors-cilostazol (Tsuchiya et al. [201]) |
Effective general therapy |
Effective in experimental models |
Ito blockers |
Quinidine (Yan and Antzelevitch [154]) |
4-aminopyridine (Yan and Antzelevitch [154]) |
β adrenergic agonist |
Isoproterenol (Yan and Antzelevitch [154]) |
Dobutamine (de la Coussaye et al. [281]) |
INa agonist |
Dimethyl lithospermate B (Fish et al. [282]) |
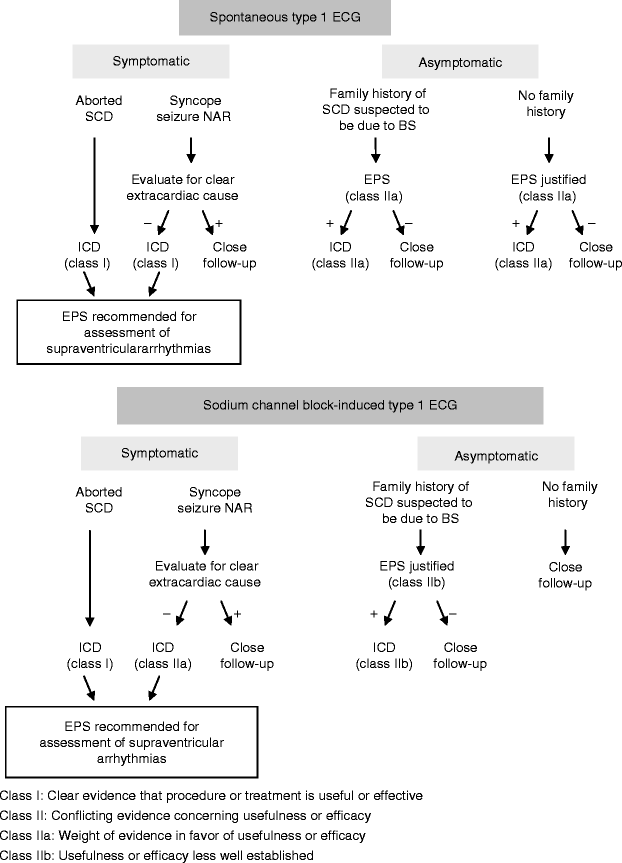
Figure 29–10.
Indications for ICD implantation in patients with the Brugada syndrome. BS Brugada syndrome, EPS electrophysiologic study, NAR nocturnal agonal respiration, SCD sudden cardiac death (From Antzelevitch et al. [10] with permission from Wolters Kluwer Health. From Antzelevitch et al. [9] with permission from Elsevier Limited)
1.
Symptomatic patients with a Type 1 ST segment elevation or Brugada ECG (either spontaneously or after sodium channel blockade) who present with aborted sudden death should receive an ICD as a Class I indication without additional need for electrophysiologic study (EPS). Similar patients presenting with related symptoms such as syncope, seizure or nocturnal agonal respiration should also undergo ICD implantation as a Class I indication after vasovagal syncope has been excluded on clinical grounds and non-cardiac causes of these symptoms have been carefully ruled out. The Task Force recommended that symptomatic patients undergo EPS only for the assessment of supraventricular arrhythmia. Electrophysiologic studies to guide quinidine therapy (that is, demonstrating that readily inducible VF at baseline becomes non-inducible after quinidine therapy) may be considered for symptomatic patients unwilling or financially incapable of undergoing ICD implantation [274].
2.
The recommendation at the time was that asymptomatic patients with a Brugada ECG (spontaneously or after sodium channel block) should undergo EPS if there is a family history of sudden cardiac death suspected to be due to Brugada syndrome. EPS was thought to be justified when the family history is negative for sudden cardiac death if the Type 1 ST segment elevation occurs spontaneously. If inducible for ventricular arrhythmia, an ICD was recommended as a Class IIa indication for patients presenting with a spontaneous Type I ST segment elevation and as a Class IIb for patients who display a Type I ST segment elevation only after sodium block challenge. More recent data have called these recommendations into question and suggest that it might be more appropriate to consider both as Class IIb indications. As discussed above, recent data seriously challenge the prognostic value of EPS for asymptomatic patients, calling for modification of these recommendations.
Although ICD implantation is the mainstay of therapy for the Brugada syndrome, implantation can be challenging in infants and is not an adequate solution for patients residing in regions of the world where an ICD is unaffordable. Also, ICD implantation in “asymptomatic high-risk patients” is not trivial, especially because the level of risk is still being debated (see Sect. “Prognosis and Risk Stratification” above). One should recall that in the Antiarrhythmics vs Implantable Defibrillators (AVID) trial, a multicenter study of ICD implantation for patients with heart disease and malignant arrhythmias, the risk for ICD-related complications serious enough to warrant re-intervention was 12 % [284]. The rate of serious complications from ICD implantation for patients with Brugada syndrome is likely to be higher than the 12 % reported for the patients in AVID, who were relatively old (65 ± 11 years) and had a 3-year mortality rate of 25 % [285]. Patients with Brugada syndrome are younger, have a very low risk for non-arrhythmic cardiac death and will remain at risk for ICD-related complications for many more years. In particular, the risk for potentially serious complications like infection or lead-insulation break leading to inappropriate ICD shocks, will increase over the years and after repeated ICD replacements. Indeed, Sacher et al. [286] reported a 28 % rate (8 % per year) of serious complications, including a 20 % risk of inappropriate shocks, in young patients with Brugada syndrome. Therefore, although the ICD represents the most effective way to prevent arrhythmic death in Brugada syndrome, pharmacologic and other solutions may be desirable as an alternative to device therapy for select cases [275] as well as for minimizing the firing of the ICD in patients with frequent events [9, 275, 287, 288].
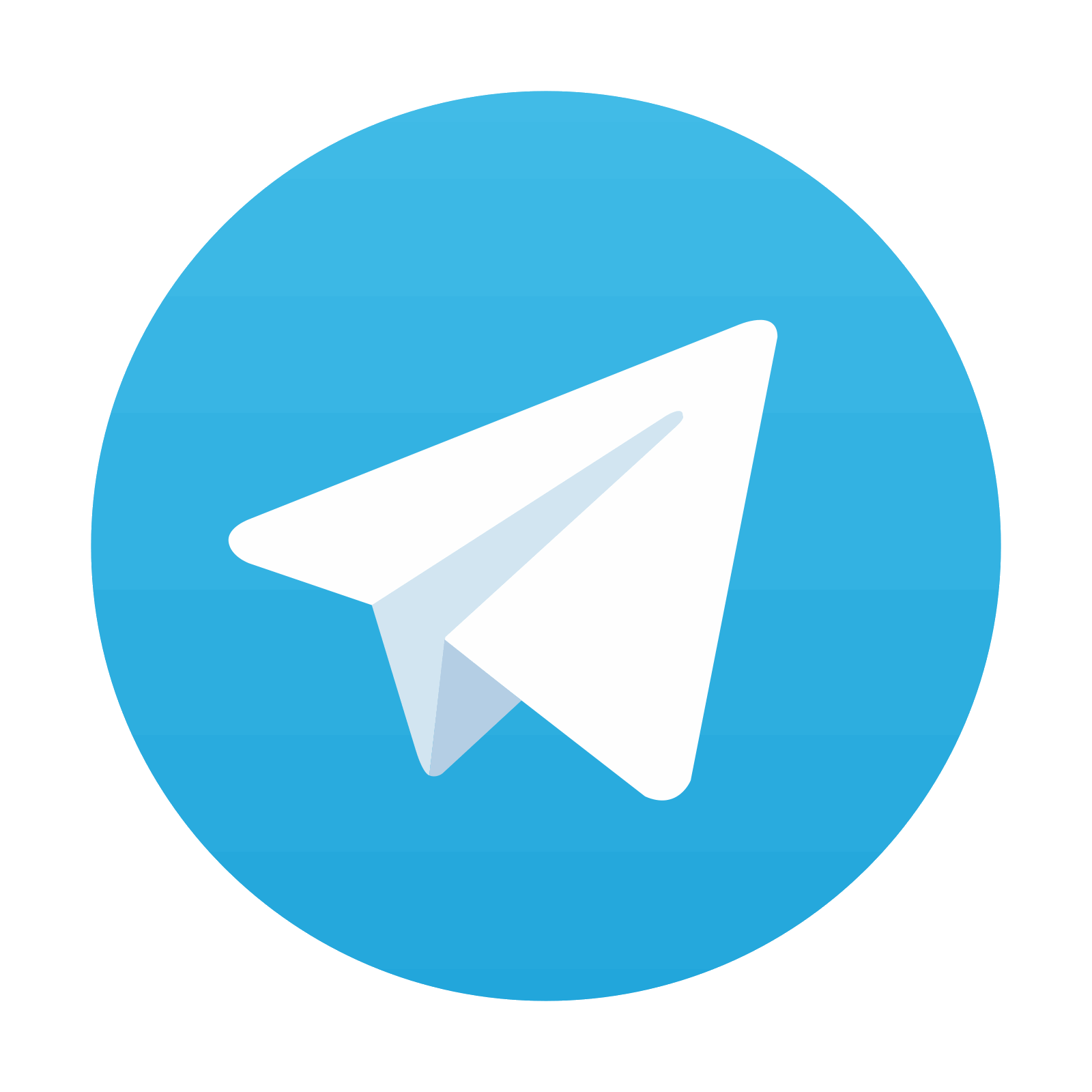
Stay updated, free articles. Join our Telegram channel
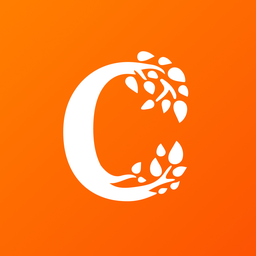
Full access? Get Clinical Tree
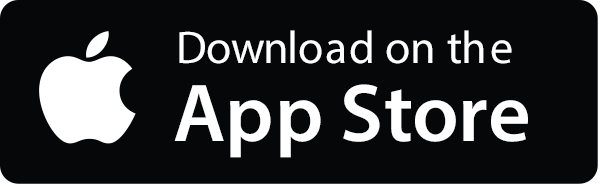
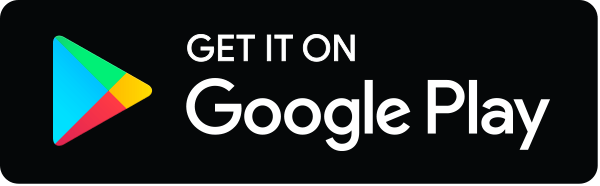