Key Points
- •
Precooling of N 2 O just before it enters the balloon catheter increases its cooling power.
- •
The elongated shape of the cryoballoon optimizes tissue contact and heat transfer.
- •
Cryoagent must be sprayed inside of the balloon in a manner maximizing cold temperatures at the equator of the balloon.
- •
The width of the equator is important to maximize lesion depth.
- •
Cryoballoon applications of 1.5-minute duration are sufficient to cause transmural lesions in animal models.
- •
270-degree curvature deflection of the sheet facilitates access to pulmonary veins.
- •
Pulmonary vein occlusion is important in maximizing cryoenergy effect and achieving transmural lesions.
Introduction
Pulmonary vein isolation (PVI) using an anatomic approach is not a new idea. Concepts such as an ultrasound saline-filled balloon, mesh, endoscopic laser ablation, high-intensity focused ultrasound, circular radiofrequency (RF) ablation catheters, and cryoballoons have been attempted. Success has ranged from marginal to equivalent success of reported PVI with RF. Cryogenic PVI is compelling because it simplifies the procedure, allows for isolation with significantly less time, has potentially equivalent outcomes of PVI with RF, and has safety benefits relative to RF.
Cryoenergy appears to have some advantages relative to RF pertaining to stroke, atrioesophageal fistula, and PV stenosis. Phrenic nerve injury is also a recognized complication of RF catheter ablation, but a frequency of phrenic nerve injury has been observed with cryoenergy application. The mechanism of phrenic nerve injury has been speculated to be associated with too distal entry into the pulmonary vein and subsequent distortion of the tissue toward the nerve, but remains to be definitively defined.
The purpose of this chapter is to address the approach that the Boston Scientific Corporation (BSC) and its subsidiary CryoCor have taken to develop a cryoballoon system. Our approach has been centered on understanding the cryolesion and what is required to make a transmural lesion. It is our belief that without understanding the biophysics of energy exchange, heat sinks, and enthalpy, a balloon design will not satisfy the efficacy requirements. Factors that can influence balloon efficacy include the cryogen (refrigerant), the delivery rate and pressure, the balloon inflation pressure, and the balloon material. This chapter is set up in two parts: First, we attempt to define those elements that contribute to formation of transmural cryolesion; then, we describe the BSC cryoballoon system.
The Making of a Transmural Cryolesion
Tissue is susceptible to cryoenergy ablation based on multiple factors. First, the cooling rate should be rapid. Second, the colder the tissue temperature, the more cell death occurs until equilibrium between intracellular and extracellular freezing exists. Multiple studies have demonstrated that different tissues have different susceptibility to temperature. Neoplastic tissue ablation requires a temperature of −50°C, whereas healthy mesenchyme tissue may be vulnerable at −20°C. Third, although the optimal duration of cold exposure varies for different types of tissue, it is clear that prolonged exposure to freezing increases tissue destruction until a steady state is achieved. Fourth, choice of an optimal rate at which the tissue is warmed may enhance tissue destruction. Last, the cryosurgery literature teaches us that cycling the temperature between freeze and thaw also increases tissue destruction. The ideal tissue treatment regimen to maximize injury would look like multiple repeats of Figure 8–1 .

The mechanisms of cell death after cryoenergy exposure have been investigated in the cryosurgery literature. The first effect is on the water within and around cells. Direct cell injury begins with structural changes in the cells and crystallization of water. The ice crystals grow until the membrane and cellular organelles are damaged, presumably by shearing caused as the ice crystals form or by compressions and distortion of intracellular organelles. This mechanism of injury usually occurs with rapid cooling to temperatures less than −20°C and almost always results in irreversible death. The second mechanism of cell damage occurs when water is withdrawn from the cells (creating a hyperosmotic environment), causing solution effects that can result in cell dehydration and solute concentration. This type of injury usually occurs with slow cooling down to around −20°C; it can be reversible under the right circumstances (e.g., temperature greater than −20°C).
Cryoenergy application to tissue does not just affect the water within and around the cells; it also has a profound effect on the vasculature. During cryoapplication, the vasculature within the tissue vasoconstricts and blood flow decreases. Once the tissue temperature freezes, the circulation stops. When the cryoenergy is removed, additional damage to the cells occurs (reperfusion injury). The permeability of the vessels within the warming tissue increases, resulting in edema and endothelial damage. Much of the endothelial damage results from platelet activation and microthrombus formation. These thrombi can result in lack of circulation or complete occlusion of smaller blood vessels, or both. Thus, the cell has little probability of recovery without a blood supply.
The last element we need to understand, then, is the composition of the cryolesion. The cells closest to the cryoenergy source achieve the coldest temperature (as well as being the fastest to cool); the farther from the source, the warmer the cells and longer it takes for them to cool. Thus, the lesion will likely have intracellular ice formation closest to the cryogenic source and more dehydration effects at the periphery of the lesion. The result may be a permanent lesion close to the cryoenergy source, a reversible region that may eventually recover farther away from the cryoenergy source, and tissue that fully recovers on rewarming yet farther from the source. This is advantageous in regard to potential for collateral tissue damage, as long as the cryoapplication is customized for the tissue thickness in which irreversible damage is desired.
Cryoenergy and its effect on cardiac tissue have been studied in preclinical models. Lower temperatures generate progressively larger lesions; however, within 5 minutes of exposure at a given temperature, the lesion size reaches plateau. Electrogram amplitude loss after cryoapplication was present acutely and persisted for 4 weeks (chronic study). Work done by Dubuc et al demonstrated that the lesion size was significantly different from 1 and 2 minutes, and between 2 and 3 minutes, but there was no difference after 3 minutes. Porcine studies have also indicated that lesions increase in size after longer treatment, but they level off at some steady-state temperature.
Knowing these effects of cryoenergy on tissue, it is now possible to consider what optimal cryoenergy application should look like in a tissue mass. We performed a numeric simulation based on an analytical model of bioheat transfer (Pennes’ equation), assuming an infinite heat sink and an idealized hollow semi-infinite spherical volume with cooling on the surface (simulation shown in Figure 8–2 A ). This theoretical modeling is a generally accepted analytical model of heat transfer in biological tissue. Figure 8–2 A shows the simulated progressive penetration of isotherms within tissue chilled by a cryoenergy source modeled after nitrous oxide (N 2 O; a computed surface temperature of −51°C). Figure 8–2 B represents the subsurface temperatures as a function of the distance from the surface of the cryoenergy source. A temperature of −20°C yields a nonreversible lesion, so by drawing a horizontal line at −20°C, the depth of the lesion can be shown to increase as the treatment time increases.

This numeric modeling teaches us two things. First, if we want to achieve intracellular ice formation with at least a −20°C isotherm (yellow in Figure 8–2 A ), then an application of 150 seconds would be required to achieve a full-thickness atrial lesion (assumes the average left atrial thickness is 2.5 ± 1.5 mm and a −51°C balloon surface temperature). The green line in Figure 8–2 A teaches us that the collateral injury from that application would be at minimum less than 3.6 mm for a treatment of 150 seconds, because healthy cells have the potential to reverse their injury at −15°C. Not surprisingly, the longer the exposure to the cryogen, the deeper the lesion (see Figure 8–2 A and Table 8–1 ). In addition, the −20°C (blue line, 300 seconds, Figure 8–2 B ) isotherm can be seen at 4 mm with a longer application compared with a 60-second application, which results in a −20°C isotherm at only 2 mm deep. However, the growth of the lesion (summarized in Table 8–1 ) slows down the deeper it penetrates; the last 1 mm takes more than 150 seconds (total time > 300 seconds), whereas the first 3 mm is achieved within 150 seconds.
DEPTH | ||||
---|---|---|---|---|
1 mm | 2 mm | 3 mm | 4 mm | |
Time for balloon surface temperature −51°C | 20 seconds | 50 seconds | 150 seconds | >300 seconds |
Time for balloon surface temperature −60°C | 10 seconds | 35 seconds | 125 seconds | 200 seconds |
In comparison, the analysis was performed with a balloon surface temperature of −60°C. Figure 8–2 C and 8–2 D show, just as in the −51°C surface temperature, that different isotherms reach specific temperature depths after specific time exposure. The colder the balloon surface, the shorter the required exposure time to reach the 3-mm tissue depth. Table 8–1 shows that the 10° difference in balloon surface temperature can create a notable difference in the time to get to a lethal temperature.
We wanted to understand whether our model was predictive of data that we could generate in tissue mimetic models. The freezing substrate was formulated using ballistic gelatin, which has properties (viscosity and density) that closely resemble human muscle tissue. The gel is optically transparent at room temperature, and a region of the gel turns permanently opaque when the gel freezes ( Figure 8–3 A , solid black line). Specifically, the gel composition was 12% formalin, 20% gelatin, and 68% water. The fixture was designed with the gel in a tapered tube configuration in the shape of the antrum of a PV (inner diameter increasing from 13 to 30 mm, with an overall wall thickness of 7 mm). Once molded and hardened, we used the mimetic as an idealized pulmonary vein structure. The surface temperature of the balloon was kept constant at −50°C, and lesions were created at four different time points. After each experimental run, the gel was sectioned and examined under a microscope to provide measurements of the scarring depth. The data are shown in Figure 8–3 . We then mapped the temperature versus depth at each time exposure (see Figure 8–3 B ). The data closely correlate with the modeling data; that is, the longer the exposure, the deeper the lesion. The offset of each lesion application increases with the longer durations (see Figure 8–3 B ). Lastly, when the gel model data are correlated with the numeric simulation data, good agreement is found between the two data sets (see Figure 8–3 C ). These results suggest that the numeric and gel tissue mimetic closely align and suggest that an application of 300 seconds will achieve a tissue depth of 4 mm.

The next question we attempted to answer was whether tissue derived ex vivo would yield the same results. To perform this experiment, we used freshly harvested porcine hearts. We performed epicardial ablation by exposing the tissue to a dry ice pellet of approximately 25-mm diameter size. We used dry ice as a surrogate to a balloon because the surface temperature achieved by the balloon approximates that which can be achieved with the sublimation of dry ice. The tissue samples were halved at the approximate center of the lesion and placed in a solution of 1% triphenyltetrazolium chloride in phosphate-buffered saline for 30 seconds. Lesion depth was assessed using digital calipers. Tissue was subsequently processed for histologic analysis, including staining the sections with standard hematoxylin and eosin ( Figure 8–4 A ). The data demonstrated that the lesion depth plateaus at 3 mm (see Figure 8–4 B ), and that when the data are overlaid with the numerical data, the lethal isotherm was located between −20° and −25°C, depending on cryoenergy exposure duration. Again, the data suggest that achieving a −20° to −25°C isotherm is sufficient to cause irreversible damage to cardiac cells. Thus, designing a balloon to achieve that temperature at 3- to 4-mm tissue depths should be sufficient to achieve PVI.

Lastly, we confirmed that the idealized vein mimetic and ex vivo cardiac ablation correlated with in vivo pulmonary vein ablation. We performed chronic porcine pulmonary vein ablations using the BSC cryoballoon system (CBAS) according to the outline in Table 8–2 . The CBAS application was applied for 1.5 and 3 minutes, and repeated twice after confirmation of vein occlusion. Figure 8–5 demonstrates a nonoccluded and occluded vein as determined by dye application and retention within the vein. The pigs were sacrificed after 40 to 46 days. Figure 8–6 depicts a step section histologic analysis of a right pulmonary vein treated with the BSC CBAS system with two applications of 3 minutes each. The tissue was sectioned in 1-mm increments and stained with hematoxylin and eosin/trichrome. Ten of 11 PVs were confirmed to be isolated after 40 to 46 days and either 2 × 1.5- or 2 × 3-minute treatment cycles. Balloon temperatures ranged from −50°C to −80°C. In addition, there was no pulmonary vein stenosis or collateral damage to the heart, lungs, phrenic nerves, and esophagus ( Table 8–3 ); however, the distance from the pig PV antrum to the phrenic nerve may be different from that in humans. Thus, the treatment of the porcine pulmonary veins with the BSC CBAS system was sufficient to cause both acute and chronic PVI, without collateral damage. These data are consistent with the hypothesis that sufficient cryo power was available to drive the −20°C isotherm to full cardiac tissue thickness, without overpowering the system to cause collateral damage by overtreatment.
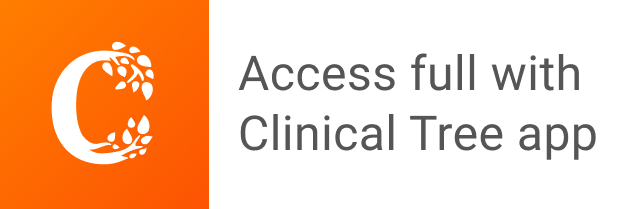