Fig. 11.1
Black blood imaging is shown in a patient referred for cardiac magnetic resonance. A right atrial mass seen by echocardiography corresponded to a prominent Eustachian valve (arrow)
A simplified pulse sequence diagram for black-blood contrast CMR is seen in Fig. 11.2. This diagram describes the time sequence of radiofrequency (RF) and gradient pulses that results in suppressed blood signal within a single phase image of the heart. This sequence of events not only suppresses signal from the ventricular cavities but also preserves signal arising from the myocardium. The pulse sequence can be separated into two distinct parts (Fig. 11.2): the so-called “double inversion” spin preparation, which occurs right after the detection of the R-wave of the EKG, and the readout, which occurs during late diastole. There are three basic concepts contributing to blood suppression in this pulse sequence. First, the intrinsic property of the fast spin echo (FSE) (a.k.a. turbo spin echo, TSE) readout to dephase moving spins and therefore reduce signal. Second, the null signal point encountered during inversion recovery. Last, the exchange of blood within the slice of interest. These basic ideas will be described below in more detail.
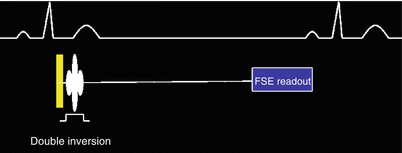
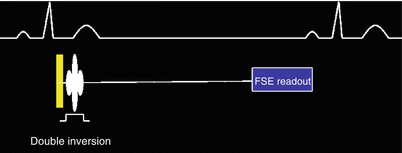
Fig. 11.2
Simplified black-blood pulse sequence
The first contribution to black-blood contrast is due to the FSE readout itself. The FSE readout, which is used in black-blood contrast, consists of a 90° excitation RF pulse (Fig. 11.3), which brings the longitudinal magnetization from the z-axis to the transverse plane so that imaging and signal acquisition can be performed. The transverse magnetization is then rephased multiple times into multiple spin echoes by a series of consecutive equidistant 180° RF pulses. This recycles the magnetization and multiple phase encoding steps can be performed from a single excitation, thus speeding up image acquisition. In theory, a single train of 180s could be used to acquire all phase encoding steps of an entire image. In practice, for cardiac imaging the length of the echo train is limited (to approximately 100–120 ms) by the T2 decay and the time period available for imaging during the cardiac cycle, which by design coincides with diastasis so that cardiac motion and related artifacts are minimized. In practice, image acquisition is segmented and the image is collected over several cardiac cycles by using echo train lengths of 12–32. The spin echoes acquired with the 180 echo train exhibit different T2 losses since the spins of the different echoes spend different amounts of time on the transverse plane. The later the echo is acquired, the more T2-weighting it carries. Carefully arranging the acquired echoes within the k-space acquisition matrix can yield proton density or T2-weighted images if the FSE readout is applied during every other heartbeat (2RR imaging). If the FSE readout is applied during every heartbeat (1RR imaging) then T1-weighted images can be obtained.
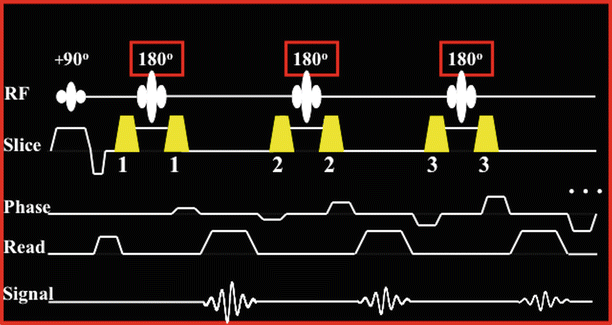
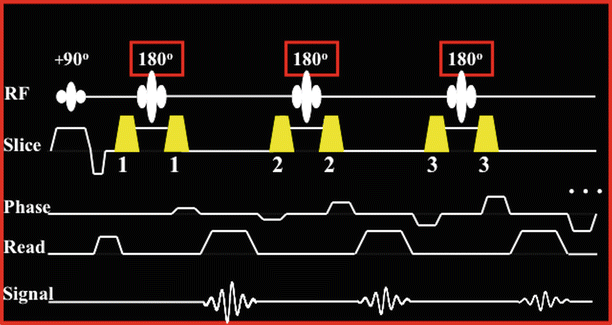
Fig. 11.3
The FSE readout used during diastasis
The FSE readout has the tendency to suppress signal from moving blood by means of through-plane motion and intravoxel dephasing. Spins excited by the 90° pulse that move outside of the imaging plane during the application of the 180° pulses will not contribute bright signal to the image. This helps create dark-blood contrast. Intravoxel dephasing occurs when the spins within a voxel of the image are not aligned and are at different angles (i.e. have different phases) with one another. This causes reduction of the signal arising from that voxel. In the most extreme case of intravoxel dephasing the spins will have phases opposite to one another and as a result zero signal will be obtained from the voxel. One can think of this as a tug of war where nobody wins. The combination of strong gradient pulses and turbulent blood motion results in spin mixing within a voxel and therefore intravoxel dephasing exists.
Figure 11.4 shows a voxel that extends from 0 to 3 on the y-axis and the effect of two gradient pulses applied along the y-axis. These gradient pulses are the same except for their opposite signs. The positive gradient pulse imparts phase to the spins depending on where they sit on the y-axis: the further away from zero, the more phase the spins acquire. If the spins are stationary then the negative gradient pulse imparts an equal phase with opposite sign to each spin thus bringing the spins into alignment and therefore maximum signal from the voxel will be acquired. However, when turbulent motion is present, as is the case with blood, the spins are likely at a different y-axis location when the negative gradient pulse is applied. For example, in Fig. 11.4, the green spin moves from position 0 to position 2 on the y-axis. Therefore, the phases from the two gradients do not cancel out and a weaker signal is acquired. If the gradients are strong enough and the blood motion is sufficiently turbulent then the spins can point in opposite directions. Then full intravoxel dephasing can occur, therefore resulting in zeroing of the signal.
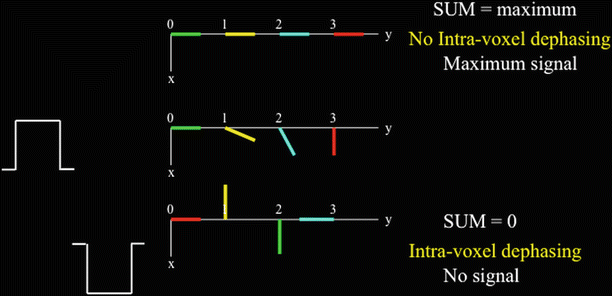
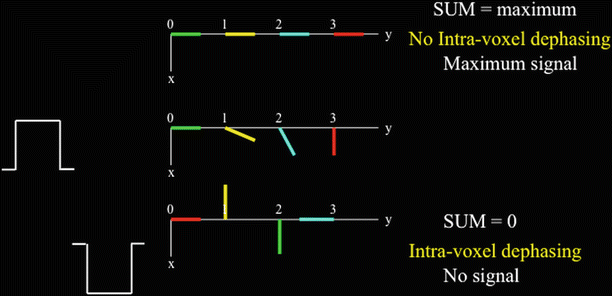
Fig. 11.4
Intra-voxel dephasing when blood spins mix (i.e. change position) during gradient pulse application
The FSE readout uses strong gradient pulse pairs that bracket the 180s (numbered gradient pulses in Fig. 11.3) so that the 180° RF pulses only refocus existing spins and do not cause new spin excitation. The details are beyond the scope of this chapter. The important concept is that moving blood spins experience these strong gradient pulse pairs during the FSE readout. Therefore, in FSE, intravoxel dephasing occurs and blood signal is suppressed to some extent. Unfortunately, blood signal suppression is not adequate and some of the moving spins can cause image flow artifacts.
The second and third contributions to black-blood contrast are due to the “null point” of the inversion recovery and due to blood motion. The so-called “double inversion” spin preparation in conjunction with through-slice blood motion further suppresses the moving blood signal in order to produce black-blood images of diagnostic quality. This preparation is applied as soon as the R-wave of the EKG is detected. Theoretically at least, it affects the exact same myocardial tissue that is imaged by the FSE readout during diastasis. This is because the spin preparation is applied prior to any cardiac contraction and because, at least theoretically, the myocardial tissue contracts and then returns to the same location in space during diastasis. This is certainly not true for the flowing blood within the cavities of the heart. It is this difference between tissue and blood that the spin preparation takes advantage of in order to preserve the signal from the myocardial tissue and suppress the signal from the blood. The actual sequence of events is described below.
< div class='tao-gold-member'>
Only gold members can continue reading. Log In or Register a > to continue
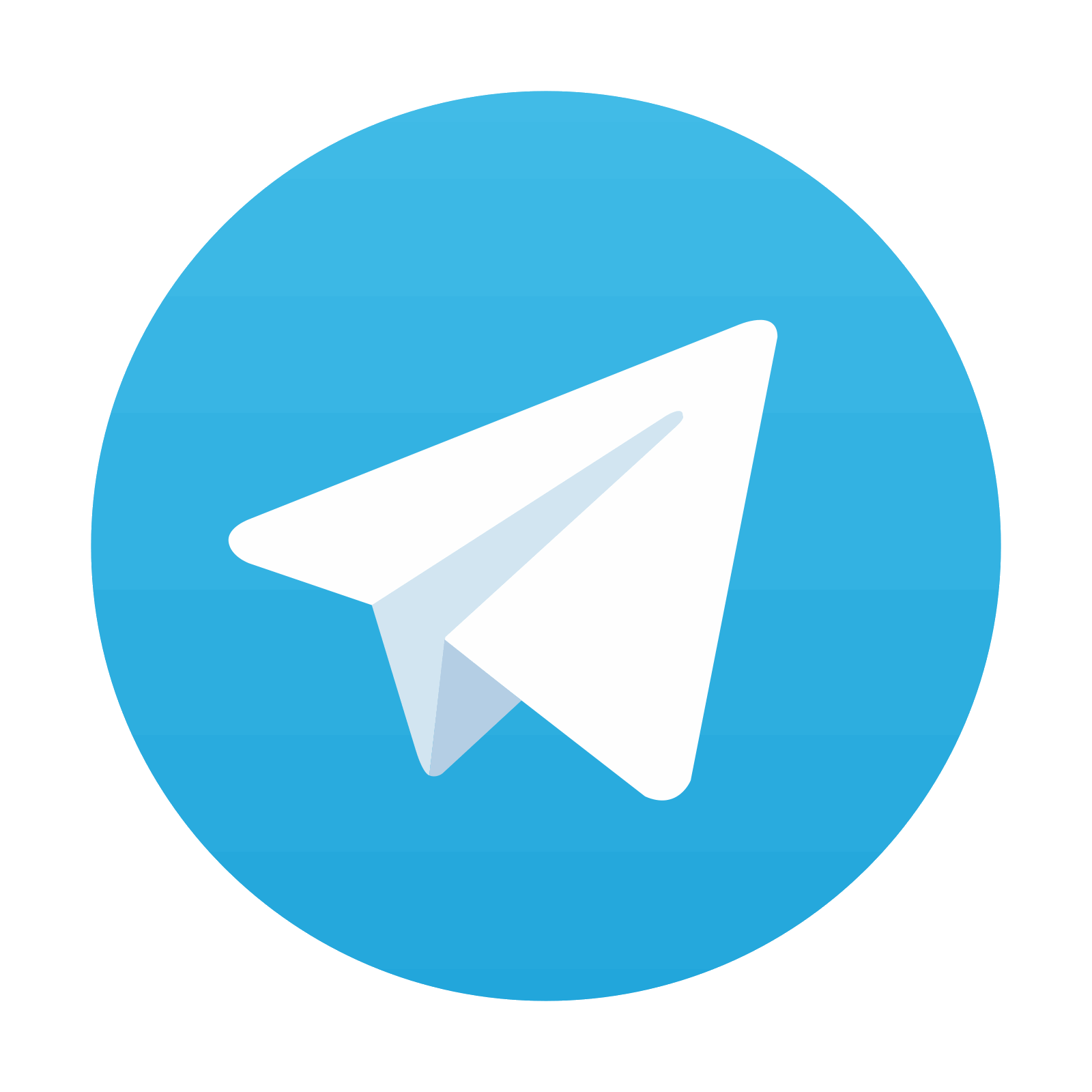