Fig. 9.1
Diagram demonstrating how proinflammatory and pro-fibrotic mechanisms impact on vascular inflammation and remodelling in vascular injury. Oxidative stress plays an important role in vascular injury. Biomarkers of these processes may provide insights into molecular mechanisms underlying vascular damage and may act as markers to predict the risk of cardiovascular disease
Many circulating biomarkers of vascular injury have been identified, including acute-phase proteins (C-reactive protein (CRP), pentraxin, amyloid A, homocysteine), inflammatory mediators (cytokines, chemokines), cellular markers of endothelial damage (microparticles, endothelial progenitor cells, microRNAs) and markers of oxidative stress (lipid peroxidation, ROS, antioxidants) [10–13]. Although the field is growing, there are still no ideal markers to predict and track vascular damage. With improved and more sensitive methodologies to measure circulating and urine biomarkers together with non-invasive vascular imaging, the domain will continue to develop, and hopefully clinically useful algorithms based on biomarkers could assist in better prediction of risk, stratification of disease and targeting of treatment. This chapter describes vascular inflammation and oxidative stress in the context of cardiovascular disease and focuses on some biomarkers that may act as clinically useful surrogates of underlying vascular injury.
9.2 Definition of a Biomarker
The term “biomarker” is a contraction of “biological marker”. An NIH working group defined biomarkers as “A characteristic that is objectively measured and evaluated as an indicator of normal biological processes, pathogenic processes, or pharmacologic responses to a therapeutic intervention” [14]. Biomarkers may be useful in the early detection of vascular injury and in predicting the risk of cardiovascular disease. Biomarkers may also reflect vascular injury and hence provide insights into mechanisms underlying progression of disease. Finally, they may act as indices to better stratify patients, which should lead to more directed and mechanism-targeted treatments. Examples of biomarkers that have been included into clinical practice and may add value to classical cardiovascular risk factors include high-sensitivity CRP for cardiovascular risk prediction, glycated haemoglobin (HbA1C) for the diagnosis of diabetes, N-terminal pro-B-type natriuretic peptide (NT-proBNP) for heart failure diagnosis, troponin I and troponin T for acute myocardial infarction diagnosis and microalbuminuria for kidney disease [15–19].
9.3 Vascular Inflammation
Low-grade inflammation in the vascular wall is increasingly recognised as an important contributor to the pathophysiology of cardiovascular disease [20], to the initiation and progression of atherosclerosis and to the development of hypertension [20, 21]. Inflammation participates in vascular remodelling and promotes accelerated vascular damage in aging through processes that involve increased expression of adhesion molecules (vascular cell adhesion molecule-1 (VCAM-1), intercellular adhesion molecule-1 (ICAM-1)) on the endothelial cell membrane, accumulation of monocytes/macrophages in the vascular wall and stimulation of ROS-generating enzymes, including Noxs [22–24] (Fig. 9.2). Innate immunity has been implicated to contribute to the low-grade inflammatory response in atherosclerosis and hypertension where different subsets of T lymphocytes may be involved in processes leading to inflammation [25–27]. For example, an imbalance exists between the proinflammatory Th1, Th2 and Th17 and the anti-inflammatory T regulatory (Treg) subsets of T lymphocytes [28].
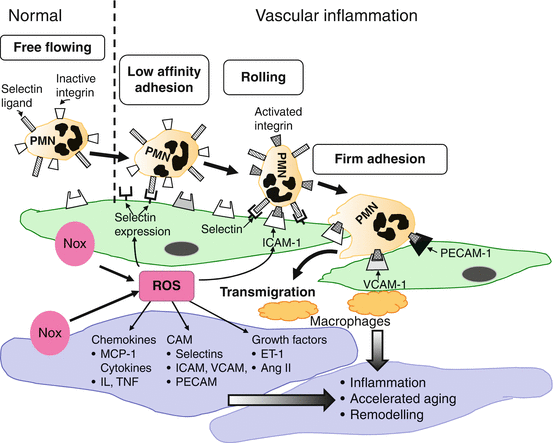
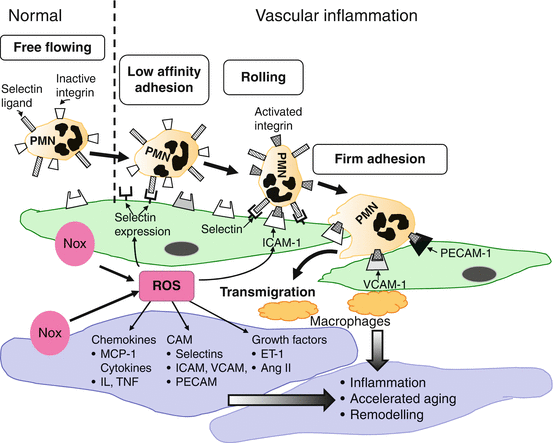
Fig. 9.2
Low-grade inflammation in the vascular wall is an important contributor to the pathophysiology of cardiovascular disease. Inflammation participates in vascular remodelling and promotes accelerated vascular damage in aging through processes that involve increased expression of adhesion molecules (vascular cell adhesion molecule-1 (VCAM-1), intercellular adhesion molecule-1 (ICAM-1), selectin, PECAM) on the endothelial cell membrane, increased rolling and adhesion of polymorphonucleocytes (PMN), transmigration of PMN and accumulation of monocytes/macrophages in the vascular wall. These processes are stimulated by increased generation of reactive oxygen species (ROS) from Noxs in endothelial and vascular smooth muscle cells (VSMC)
9.4 Reactive Oxygen Species, Oxidative Stress and Vascular Inflammation
ROS are formed during the reduction of oxygen and include unstable free radicals (species with an unpaired electron) such as superoxide (O2 •−) and non-free radicals, such as hydrogen peroxide (H2O2) [29]. ROS are generated continuously as normal by-products of cellular metabolism and as direct products through activation of nicotinamide adenine dinucleotide phosphate (NADPH) oxidase, also called Nox [30, 31]. ROS are produced in the endothelium, throughout the vascular wall and adventitia and in perivascular fat. In the vascular system together with nitric oxide (NO), a potent endothelial-derived vasodilator, ROS play a physiological role in regulating endothelial function and vascular contraction/dilation and are important in maintaining vascular integrity [32–34]. Under pathological conditions, increased ROS levels cause cell damage and promote vascular inflammation, hypertrophy, fibrosis and increased contraction, important factors contributing to endothelial dysfunction and vascular remodelling in cardiovascular diseases [35, 36]. Molecular processes underlying ROS-induced cardiovascular injury involve activation of redox-sensitive signalling pathways [37–39]. Superoxide anion and H2O2 stimulate mitogen-activated protein kinases (MAPK), tyrosine kinases, Rho kinase and transcription factors (NFκB, AP-1 and HIF-1) and inactivate protein tyrosine phosphatases (PTP) [40, 41]. ROS also increase intracellular free Ca2+ concentration ([Ca2+]i) and upregulate proto-oncogene and proinflammatory gene expression and activity [42]. These phenomena occur through oxidative modification of proteins [43]. Changes in the intracellular redox state through glutathione and thioredoxin systems may also influence intracellular signalling [44].
9.4.1 Production and Metabolism of ROS in the Cardiovascular System
Enzymatic sources of ROS important in cardiovascular disease are xanthine oxidoreductase, uncoupled NO synthase (NOS), mitochondrial respiratory enzymes and NADPH oxidase [45–47], found in many cell types in the heart, kidney, vessels and central nervous system. Of the many ROS-generating enzymes, it is only NADPH oxidase that has as its primary function the formation of ROS, hence termed a “professional” ROS producer [30, 31]. NADPH oxidase was originally considered to be expressed only in phagocytic cells involved in host defence and innate immunity. It is now evident that there is a family of NADPH oxidases/Noxs that are functionally active in non-phagocytic cells, including vascular cells. The mammalian Nox family comprises seven members: Nox1, Nox2, Nox3, Nox4, Nox5, Duox1 and Duox2 [48, 49]. All are transmembrane proteins that have conserved structural properties and that transport electrons across biological membranes to reduce O2 to O2 •−. Each isoform is encoded by separate genes. Whereas Nox1, Nox2, Nox4 and Nox5 have been identified in cardiovascular tissue [50], Nox3, found in the inner ear, and Duox1 and Duox2, found primarily in the thyroid gland, do not seem to be important in the cardiovascular system.
9.5 Biomarkers of Endothelial Dysfunction
9.5.1 Nitric Oxide (NO) and ROS
Biomarkers of impaired endothelial function reflect altered NO bioavailability, increased oxidative stress, coagulation and endothelial inflammation. NO, produced by endothelial cells, is a major determinant of endothelium-dependent vasodilation and is an inhibitor of coagulation, inflammation and oxidative stress [51–53] and consequently has been considered as an important marker reflecting endothelial status. Since NO has a short half-life, plasma levels of oxidative degradation products of NO, including nitrite (NO2 −), nitrate (NO3 −) and nitrosothiols, have been used as surrogate indices of NO generation [54]. In addition to assessing levels of NO and its metabolites, measurement of asymmetric dimethylarginine (ADMA), a potent endogenous inhibitor of nitric oxide synthase (NOS)-derived NO production, could also reflect NO bioavailability [55]. Plasma ADMA levels have been shown to correlate with endothelial NOS activity and to be associated with endothelial dysfunction [56, 57].
Endothelial injury is associated with increased production of O2 •−, which readily reacts with NO to form peroxynitrite (ONOO−), an injurious free radical that further contributes to endothelial dysfunction [58]. Similarly, lipid peroxyl radicals react with NO and may also be a source of NO inactivation [59]. Plasma measures of endothelial oxidative stress include indices of lipid peroxidation (F2-isoprostanes and thiobarbituric acid-reactive substances) and nitrotyrosine levels, reflecting peroxynitrite generation [60, 61]. Lipid peroxidation is increased in diseases associated with endothelial dysfunction, and there is a strong negative correlation between oxidative stress and endothelial function [62–64].
9.5.2 Endothelial Proinflammatory Markers
Because endothelial impairment is often accompanied by inflammation, proinflammatory markers have been used to reflect dysfunction. Cellular adhesion molecules play an important role in endothelial inflammation by attracting and anchoring inflammatory cells. Soluble forms of cell adhesion molecules including VCAM-1, ICAM-1 and E-selectin are found in the circulation and may reflect vascular inflammatory status and endothelial dysfunction [65]. C-reactive protein, a protein found in plasma, which rises in response to inflammation, is an independent predictor of abnormal endothelial function and cardiovascular mortality and morbidity [66, 67]. Additional circulating markers of inflammation include soluble CD40L [68] and circulating cytokines such as interleukin-6 and tumour necrosis factor-α (TNFα) [69].
9.5.3 Markers of Coagulation
Endothelial dysfunction is associated with a pro-coagulatory state and accordingly indices of coagulation may be considered as biomarkers of endothelial status. Plasminogen activator inhibitor-1 (PAI-1) is an inhibitor of fibrinolysis that is produced primarily by the endothelium [70]. P-selectin is a platelet activation marker and plasma fibrinogen levels are thought to be reflective of a pro-coagulant state. PAI-1, P-selectin and fibrinogen are increased in cardiovascular disease and correlate with endothelial dysfunction [71]. Von Willebrand factor (vWF), a glycoprotein which is secreted by endothelial cells and plays a role in coagulation, has also been proposed as a biomarker of endothelial function [72].
9.5.4 Microparticles
In addition to circulating biochemical or protein-based markers, growing evidence indicates that endothelial cell-derived fractions, including microparticles, may be reflective of endothelial dysfunction and injury. Microparticles are anuclear fragments of cellular membrane shed from stressed or damaged cells [73]. They are typically 0.1–1.0 μm in diameter and contain surface proteins and cytoplasmic material of the parent cells. Microparticles are distinguishable from other subcellular vesicles, such as exosomes and apoptotic bodies, on the basis of size, mechanism of formation and content [74].
Microparticles are identified in plasma samples by flow cytometry on the basis of size, the externalisation of phosphatidylserine and the presence of specific surface antigens. Labelling of surface antigens allows for identification of the parent cell from which the microparticles were derived. In plasma samples, microparticles of endothelial (identified by the surface presence of CD144, CD62E or CD31), platelet (CD41a, CD42b, CD62P), leukocyte (CD45, CD4, CD8, CD14) and erythrocyte (CD235a) origin are present [75–77]. Given that microparticles are released under conditions of cell stress/damage, it is not surprising that plasma levels of microparticles are increased in cardiovascular disease. Strong evidence exists to suggest that microparticles of endothelial, platelet and leukocyte origin may be reflective of endothelial dysfunction and may in fact contribute to endothelial dysfunction [78–80]. Endothelial microparticles are thought to be directly indicative of endothelial cell stress/damage and may also reflect endothelial inflammation, increased coagulation and vascular tone [81]. Platelet microparticles may indirectly reveal endothelial dysfunction by revealing increased coagulation and inflammation [82], while leukocyte microparticles may reflect inflammation, coagulation and vascular tone [83]. Microparticles of endothelial, platelet and leukocyte origin have been shown to be increased in multiple disease states associated with endothelial dysfunction including hypertension, diabetes and end-stage renal disease (reviewed in [84]). Considering the complexity of endothelial function, measuring multiple biomarkers to reflect different processes may provide a comprehensive assessment of endothelial status.
9.6 Biomarkers of Vascular Inflammation
9.6.1 Acute-Phase Response Proteins
Acute-phase proteins are a class of proteins, which are increased in response to inflammation. The phenomenon is called the acute-phase reaction [85]. Acute-phase proteins are produced by the liver in response to injury and under the control of a cascade of cytokines including IL-1β, IL-6 and TNFα. The association of elevated serum levels of acute-phase proteins with the progression of atherosclerosis, coronary artery disease, unstable angina and myocardial infarction has been documented in epidemiological studies [86, 87]. Acute-phase protein markers including CRP, pentraxin 3 (PTX3), amyloid A, homocysteine and fibrinogen are increased in cardiovascular disease and reflect generalised inflammation.
9.6.1.1 C-Reactive Protein
CRP has been the plasma biomarker of choice for a clinical index of generalised inflammation partly due to its wide dynamic range. Increased plasma CRP has been demonstrated in many cardiovascular diseases associated with endothelial dysfunction and vascular injury. Tissue necrosis is a potent inducer of CRP, and in post-myocardial infarction, there is a significant CRP response [88]. The Emerging Risk Factors Collaboration evaluated the predictive risk of adding CRP, fibrinogen and lipid-related markers (apolipoprotein A, apolipoprotein B, apolipoprotein A-1, lipoprotein(a) or lipoprotein-associated phospholipase A2) to traditional risk factors in a meta-analysis including over 240,000 participants with no history of cardiovascular disease [89]. Adding either of these biomarkers resulted in only a modest reclassification improvement for individuals at intermediate risk versus models that included age, sex, smoking, blood pressure, diabetes and total cholesterol concentrations. CRP failed to predict incident cardiovascular disease in patients with diabetes [90]. Although initial experimental evidence suggested that CRP might itself be pro-atherogenic and cause vascular disease, later studies refuted this.
9.6.1.2 Other Acute-Phase Proteins
Besides CRP, there are other acute-phase proteins that are increased in cardiovascular disease. Pentraxin 3 appears to be more specific for vascular inflammation than CRP [91]. In patients with stable coronary artery disease, increased PTX3 levels were associated with increased risk for all-cause mortality, cardiovascular events and heart failure [92]. Elevated serum levels of homocysteine and cardiac troponins are biomarkers of myocardial injury and are elevated in patients with cardiac failure [93, 94]. Raised plasma homocysteine is associated with greater risk for more severe cardiovascular disease. Increased concentrations of plasma amyloid A and fibrinogen are linked to a large number of cardiovascular risk factors and have been associated with increased risk for coronary artery disease, thrombosis and myocardial infarction [95].
9.6.2 Cytokines
9.6.2.1 Tumour Necrosis Factor-α (TNFα)
Tumour necrosis factor-α and interleukins are proinflammatory cytokines that are upstream of the acute-phase response and are thus generally thought to be more credible causal agents in atherosclerosis and CVD. Cytokines are soluble glycoproteins produced by many cell types including cells of the vascular and immune systems [96]. They are produced in response to tissue injury or ischaemia and are responsible for controlling the innate and adaptive immune response. An increase in production of proinflammatory cytokines, such as IL-1β, IL-6 and TNFα, and a decreased production of anti-inflammatory cytokines, such as IL-10, have been observed in many diseases associated with endothelial dysfunction and vascular injury, including hypertension, heart disease, stroke and diabetes [97, 98]. As such these cytokines have been implicated as therapeutic targets and biomarkers for disease progression and prognosis.
The biology of the TNFα axis is complex and its physiological roles are still being elucidated. The TNFα precursor is membrane bound and cleaved by TNFα-converting enzyme (TACE), releasing the soluble form that binds to TNFR1 and TNFR2, expressed in most cell types [99]. TNFα signalling via TNFR1/TNFR2 elicits signalling for cell death, survival or inflammation by recruiting specific adaptor proteins. Recruitment of adaptor proteins such as caspase-8 leads to apoptosis, while recruitment of TNF receptor-associated factor 2 (TRAF2) and receptor-interacting protein (RIP) stimulates signalling through NFκB resulting in activation of transcription factors and genes that promote inflammation [100].
9.6.2.2 Interleukin-1β (IL-1β)
Interleukin-1β is constitutively expressed in low levels and is regulated by transcription, translation, cleavage and cellular release [101]. It is synthesised as a large precursor protein and is biologically inactive until it is secreted following cleavage by caspase-1. Active IL-1β binds to IL-1R1, to induce proinflammatory signalling by stimulating Ca2+ channel activation, increasing expression of adhesion molecules on endothelial cells and causing impaired endothelial permeability [102].
9.6.2.3 Interleukin-6 (IL-6)
Interleukin-6 is an inflammatory cytokine that binds to class 1 cytokine receptors, inducing activation of Janus kinase (JAK), which induces activation of signal transducer and activator of transcription (STAT) family of transcription factors and RAS-RAF-MAPK pathways, leading to proinflammatory responses [103].
Increased plasma levels of IL-6 and TNFα have been demonstrated in patients with diabetes, hypertension, ischaemic heart disease and cardiac failure [104–106]. In a large prospective population-based study, a combined elevation of IL-1β and IL-6 was independently associated with an increased risk of type 2 diabetes, suggesting a role for low-grade inflammation in diabetes (EPIC) [107]. However data from the large ADVANCE clinical trial challenge the inflammatory paradigm of microvascular disease in diabetes, showing that circulating cytokines are actually not strongly associated with microvascular risk after adjusting for confounding risk factors [108].
If indeed IL-6 and TNFα are implicated in the pathogenesis of vascular inflammation and associated cardiovascular disease, then treatment targeting these cytokines should reduce cardiovascular risk. Findings from studies where anti-IL-6 and anti-TNFα biologics have been used in patients with inflammatory disease such as rheumatoid arthritis suggested reduced risk of cardiovascular disease in these patients who are at −50 % increased risk of cardiovascular disease compared to the general population [109, 110]. Data from biologics treatment registers of rheumatoid arthritis patients show that those who respond to TNF biologics have reduced rates of myocardial infarction (3.5 per 1,000 person-years) compared to nonresponders (9.4 per 1,000 person-years) [111]. Similar data have been reported in the US CORRONA database [112]. It should be highlighted that these findings are based on pharmacoepidemiology and are therefore subject to potential confounding factors. Meta-analysis of small trials failed to show a significant reduction in cardiovascular risk associated with anti-TNFα biologics [113].
9.7 Biomarkers of Oxidative Stress
Because of the key role of oxidative stress in vascular injury and cardiovascular pathology, ROS levels and redox state have been considered as promising biomarkers indicative of the disease process. However, free radicals have a very short half-life and are unstable. Hence accurately measuring O2 •− and H2O2 in the circulation is complex. As such, methods have been developed to measure stable markers of ROS that reflect oxidative status. Biomarkers of oxidative stress that are currently assessed are measures of oxidation products of lipids, DNA and protein [114] (Table 9.1).
Table 9.1
Biomarkers of oxidative stress
Lipid peroxidation |
Malondialdehyde |
F2-isoprostanes |
4-Hydroxynonenal (4-HNE) |
OxLDL |
Pro-oxidant enzymes |
Xanthine oxidase |
Mitochondrial oxidases |
NADPH oxidases |
Antioxidant enzymes |
Superoxide dismutase |
Catalase |
Glutathione peroxidase |
GSH/GSSG ratio (thiol index) |
Nonenzymatic antioxidants |
Total antioxidant capacity |
Vitamin C |
Vitamin A |
Urate |
Bilirubin |
Thiols |
Flavonoids |
Carotenoids |
Protein/DNA oxidation |
Carbonyls |
8-Oxo-7,8-dihydroguanine |
Reactive oxygen/nitrogen species |
H2O2 |
NO |
ONOO− |
Cell-based biomarkers |
Microparticles |
Endothelial progenitor cells |
MicroRNAs |
9.7.1 Lipid Peroxidation
Lipids are highly sensitive to oxidation because of their molecular structure, which is characterised by numerous reactive double bonds. Polyunsaturated fatty acids, including phospholipids, glycolipids and cholesterol, are vulnerable targets of oxidation. For instance, evidence suggests that oxidised LDL (oxLDL) is engulfed, via TLR-4, by activated macrophages, which then become cytokine-producing lipid-laden foam cells, an integral part of the atherogenic plaque. Increased ROS bioavailability triggers the process of lipid peroxidation. The most commonly studied markers of lipid peroxidation are malondialdehyde (MDA) and isoprostanes.
9.7.1.1 Malondialdehyde
Malondialdehyde is the by-product of the arachidonate cycle and a main aldehyde product of lipid peroxidation in vivo. It is formed by peroxidation of polyunsaturated fatty acids and can interact with proteins [115]. MDA can be detected by thiobarbituric acid (TBA) using a colorimetric method based on the reaction between MDA and TBA that gives a pink colour [116]. The products that are measured are termed TBA-reactive species (TBARS). The TBARS assay is amongst the most widely employed to evaluate lipid peroxidation. This assay has been extensively used as an indicator of oxidative stress in experimental and human cardiovascular disease. Plasma TBARS levels are increased in patients with coronary artery disease, hypertension, atherosclerosis, diabetes, heart failure, stroke and aging [117–120]. Cigarette smokers also have elevated levels of TBARS suggesting that pro-atherogenic and vascular injury effects of smoking are related to oxidative damage induced by lipid peroxidation [121, 122]. Some studies showed that plasma TBARS could predict cardiovascular events. For example, raised TBARS concentrations predicted carotid atherosclerotic plaque progression over a 3-year period as validated by carotid wall thickness using ultrasound [123].
9.7.1.2 Isoprostanes
F2-isoprostanes are prostaglandin-like compounds produced by nonenzymatic peroxidation of arachidonic acid, a polyunsaturated fatty acid in phospholipids of cell membranes [124]. Formation of isoprostanes is independent of the cyclooxygenase enzyme that catalyses the production of prostaglandins. F2-isoprostanes are stable end products of lipid peroxidation and can be measured in all human tissues and biological fluids, including urine, plasma and cerebrospinal fluid [125]. A metabolite of F2-isoprostanes, 8-iso-prostaglandin F2α (8-iso-PGF2α), has vasoconstrictor, cell-growth properties and platelet aggregation and as such is biologically active independently of its biomarker status. F2-isoprostanes can be assessed by gas chromatography-mass spectrometry, liquid chromatography-mass spectrometry, enzyme-linked immunosorbent assay (ELISA) and radioimmunoassay in plasma and urine [126]. Levels of F2-isoprostanes in plasma and urine correlate with ROS levels and oxidative stress in experimental and human studies [127].
Increased circulating and urine levels of F2-isoprostanes have been demonstrated in various cardiovascular diseases associated with vascular injury, including hypertension, atherosclerosis, ischaemia-reperfusion injury and cardiac failure [128–130]. In addition, in healthy individuals with risk factors, such as obesity, hyperlipidaemia and hyperhomocysteinaemia, plasma concentrations of F2-isoprostanes are elevated, suggesting that indices of lipid peroxidation may be clinically relevant biomarkers of cardiovascular risk. In support of this, a recent prospective study including 1,002 anticoagulated patients with atrial fibrillation studied over 25 months demonstrated that 8-iso-PGF2α and sNOX2-dp (a marker of Nox2 levels) correlated with cardiovascular events [131]. Using various modelling paradigms, it was shown that 8-iso-PGF2α predicted cardiovascular events and death. As such it was suggested that F2-IsoP may complement conventional risk factors in prediction of cardiovascular events.
9.7.1.3 4-Hydroxynonenal
4-Hydroxynonenal (4-HNE), an α,β-unsaturated hydroxyalkenal produced by lipid peroxidation in cells, is generated during physiological and pathophysiological conditions based on the production of ROS [132]. 4-HNE is considered as one of the most specific and sensitive measures of lipid auto-oxidation. Various methods have been developed to measure 4-HNE including HPLC and GC-MS, anti-HNE adduct antibodies and ELISA.
Higher circulating 4-HNE levels have been shown to correlate with more severe diastolic dysfunction in spontaneously hypertensive rats [133]. 4-HNE has also been associated with stroke, ischaemia-reperfusion injury and cardiac hypertrophy. Increasing experimental evidence indicates that 4-HNE may have a dual role in that it may be a marker of systemic oxidative stress and also contribute directly to the pathogenesis of cardiovascular disease [134, 135]. Despite experimental evidence linking 4-HNE and cardiovascular disease, there is a paucity of information in humans. A few studies in dialysed patients demonstrated that MDA and 4-HNE thiols correlated with severity of cardiovascular disease [136, 137].
9.7.2 Nonenzymatic Total Antioxidant Capacity
Total antioxidant capacity is a measure of the combined antioxidant effect of the nonenzymatic defences in biological fluids and does not take into account the enzymatic antioxidant systems such as superoxide dismutase, catalase, peroxidase, etc. The assay measures low molecular weight antioxidants, both water soluble and lipid soluble, and includes urate, bilirubin, vitamin C, thiols, flavonoids, carotenoids and vitamin E [138]. Experimental and clinical studies have shown, for the most part, low levels of total antioxidant capacity in various cardiovascular diseases [139–141]. Total antioxidant capacity assays have also been used extensively in human studies evaluating effects of dietary antioxidants.
A recent comprehensive study reviewed prospective cohort studies and clinical trials relating to associations between plasma/dietary antioxidants (total antioxidant capacity) and cardiovascular events [142, 143]. In long-term, large-scale, population-based cohort studies, higher levels of total antioxidant capacity were associated with a lower risk of cardiovascular disease supporting a protective effect of dietary antioxidant vitamins, carotenoids and polyphenols. However results from large randomised controlled trials failed to support long-term use of single antioxidant supplements for cardiovascular prevention due to their lack of benefit or even adverse effects on major cardiovascular events or cancer. Although antioxidant supplement use was reported to have no benefit on cardiovascular events by several large randomised controlled trials, cohort studies still supported the protective effects of dietary antioxidants in preventing cardiovascular disease. In particular antioxidant vitamins and polyphenols exhibit high antioxidant capacity in vitro and cardioprotective effects in vivo. Although total antioxidant capacity assays may shed light on dietary antioxidant effects in specific patient cohorts, there are still technical concerns regarding specificity and sensitivity of these assays, and as such, total antioxidant capacity of foods or populations should not yet be used for making decisions affecting population health [144].
9.7.3 Oxidative Modification of DNA and Proteins
DNA and proteins are highly susceptible to modifications by changes in the redox state. Protein oxidation is the process whereby amino acid residues of a protein act as electron donors and suffer an oxidative attack by an oxidising agent. This process usually leads to changes in the biological function of the protein. Oxidation of proteins can be induced by various mechanisms/stimuli including ROS, metal cations, γ-irradiation, UV light and ozone, leakage of the electron transport chain in mitochondria, oxidoreductase enzymes, products of lipid peroxidation as well as activated phagocytes [145, 146].
Protein oxidative modifications can be divided into reversible, by biological antioxidant systems, and irreversible [147]. Irreversible modification normally leads to loss of protein functionality, aggregation and consequent degradation, while reversible modification has regulatory functions. The most common type of irreversible modification is the formation of carbonyl groups. Amino acids prone to carbonylation include proline, arginine, threonine, lysine, histidine and cysteine. Assessment of the extent of such a general type of oxidation serves as a marker of increased oxidative stress [148]. On the other hand, the sulfur (S)-containing amino acids, Cys and Met, are the only amino acids which can undergo modifications that can be reversed by cellular enzymes and have, therefore, a potential regulatory role in redox signalling [147]. The most common types of oxidative modifications include nitrosylation, S-glutathionylation, sulfenylation and carbonylation [149]. Another biomarker of oxidative stress is the extent of oxidation of bases in DNA. 8-Oxo-7,8-dihydroguanine or the corresponding nucleoside is most often measured, either chromatographically or enzymatically [150], as an index of DNA oxidation.
Oxidatively modified proteins regulate the activities of several redox-sensitive proteins involved in cardiovascular homeostasis, including endothelial function, myocyte contraction, vasodilation, oxidative phosphorylation, protein synthesis and glycolytic metabolism [151, 152]. At the same time, they may serve as biomarkers of oxidative stress.
Strategies to examine protein oxidation can be classified into direct and indirect assays. Direct approaches detect oxidation by exploiting the properties of oxidised forms of proteins or structural changes induced by oxidation and use specific antibodies [153, 154] or chemoselective probes [155]. The development of cell-permeable probes provides the advantage of labelling within the system, thus preventing any artefactual labelling due to oxidation upon cell lysis [155]. Indirect approaches utilising labelled substrates are the most commonly used, as they exploit conserved biochemical properties of the reversible oxidative modifications, as well as the catalytic activity of certain proteins.
While there is growing interest in studying oxidative modifications of proteins in cardiovascular tissue in experimental models, such approaches are not yet used in the clinical setting [156, 157]. However recent studies have shown that in patients with post-acute myocardial infarction, circulating levels of fibrinogen carbonylation, a marker of modified protein structure and altered fibrinogen function, are increased compared to controls [158].
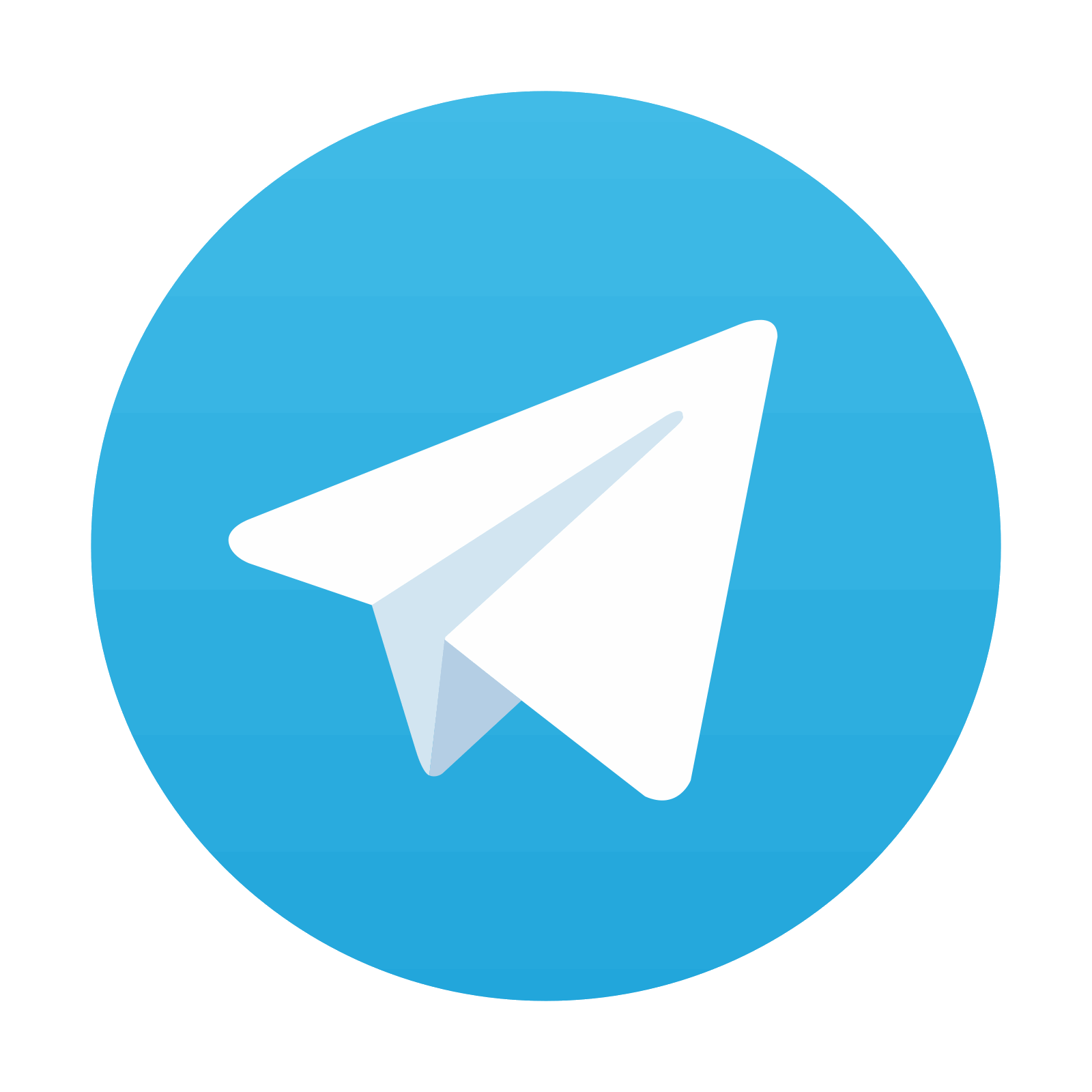
Stay updated, free articles. Join our Telegram channel
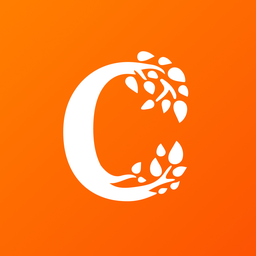
Full access? Get Clinical Tree
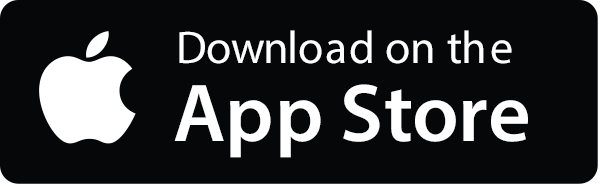
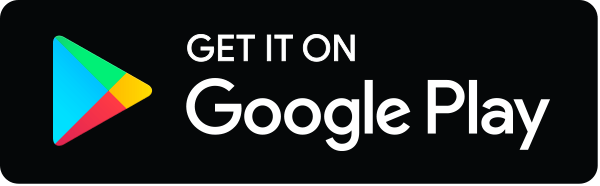