“No monitoring device can improve patient-centered outcomes unless it is coupled to a treatment that improves outcome. ” Michael Pinsky
Introduction
The Danish anesthetist Bjørn Ibsen first pioneered the intensive monitoring of critically ill patients during the poliomyelitis epidemic in the early 1950s. Yet it was not until the organization of coronary care units in the 1960s that hospitals formally adopted comprehensive monitoring of acutely ill patients safely outside the operating room setting. These units (much like today) offered continuous monitoring and aggressive interventions to improve survival in patients with acute myocardial infarction. The eventual application of this model to the care of noncardiac critically ill patients lent toward its incorporation into the core principles of intensive care medicine and set the standard for patient care in subsequent years.
The word “monitor” derives from the Latin word monere , meaning “to advise” or “to warn.” In 1984 The Monitoring of The Critically Ill Conference expanded on this concept to include “making repeated or continuous observation or measurements of the patient, his or her physiological function, and the function of the life-support equipment, for the purpose of guiding management decisions, including one to make therapeutic interventions, and an assessment of those interventions.” 3 Though others were later introduced, this definition highlights the intimate relationship between patients (humans) and monitors (machines). It is from this foundation that hemodynamic monitoring now stands as a central foundation of clinical practice in the intensive care unit (ICU).
In the modern era intensive care medicine is now nearly synonymous with intensive cardiovascular monitoring. Still it remains worthwhile to mention that the elemental task of effective hemodynamic monitoring is the early detection of cardiovascular insufficiency and its clinical presentation as “circulatory shock.” Shock is a life-threatening condition. It results in an uncoupling between tissue oxygen supply and demand, impairs cellular oxygen utilization, and leads to multiorgan failure if untreated. Common physical assessments for the presence and degree of clinical shock lack the immediacy and specificity needed to make accurate care decisions. By the time that common signs and symptoms of shock (such as hypotension, poor renal output and skin perfusion, and mental status changes) are present, important opportunities to intervene on a patient’s behalf are missed. Therefore, early recognition of the syndrome of shock is paramount to acceptable outcomes that are achievable only with close mechanized monitoring of the hemodynamic system.
High fidelity and quality monitoring require defining specific parameters that accurately represent differences between healthy and diseased states. Individualized monitoring parameters must also cater to the specific needs of specific patient populations. For example, circulatory shock in post cardiac arrest patients, or patients with hepatic failure, or patients with recent neurological injury require different therapeutic approaches; the choice of monitoring modality is dependent on these needs. Disease progression also dictates prescribed monitoring techniques. Providers using less invasive methods for patients in mild cardiovascular insufficiency states must deftly escalate to more invasive monitoring for patients progressing through their disease course. Finally, it is important to highlight that process-specific monitoring methods effectively influence therapeutic strategies and allow for a basic understanding of cardiovascular physiology to guide bedside management decisions.
Invasive Methods of Hemodynamic Monitoring
Arterial Blood Pressure Monitoring
Arterial blood pressure is an essential hemodynamic parameter in the ICU and reflects the interplay between vasomotor tone and cardiac output. Noninvasive blood pressure measurement by blood pressure cuff is, of course, a cornerstone of traditional patient vital sign assessments. Invasive arterial catheterization, a direct measurement of arterial blood flow, extracts data from the continuous monitoring of blood pressure. The arterial line pressure transducer setup links an independent catheter inserted into an accessible artery with a transducer via a fluid-filled tubing system. This allows for the conversion of pressure changes within the transducer into electrical signals that are displayed on a monitor with a waveform accompanied by numerical values. As with other monitoring modalities, this transducer system requires careful instruction in meticulous priming, dampening assessment, zeroing, and leveling to ensure the interpretation of accurate data. With proper interpretation, the waveform tracing from the invasive arterial line can yield a treasure of clinical information regarding cardiovascular health. It offers nuanced and real-time insights into the cardiac cycle, including measurements of the systolic and diastolic blood pressure, mean arterial pressure (MAP), stroke volume, and pulse pressure.
The arterial pressure waveform represents a systolic and diastolic flow of blood through the cardiovascular system with each cardiac cycle ( Fig. 4.1 ). It starts with a systolic upstroke after ventricular depolarization, manifested as the R wave on the electrocardiogram. Ventricular contraction results in a steep and positive deflection in the arterial blood pressure waveform culminating in peak systolic pressure. This segment is dependent on both the rate of change in left ventricular pressure and aortic valve integrity as different flow rates will create different slopes. For example, a patient with severe aortic stenosis has a delayed systolic upstroke segment. The decay in pressure after peak systolic pressure represents a decrease in stroke volume from an emptying left ventricle as it approaches diastole. The dicrotic notch, or “incisura,” occurs during this pressure decay and represents a brief, but abrupt, increase in blood pressure from aortic valve closure. This marks the end of systole. Arterial reservoir pressure and elastic recoil of the large arteries, in addition to peripheral vascular resistance, contribute to the gradual decline in blood pressure until it reaches the end of diastole. Peripherally vasodilated patients (as seen in individuals with hypovolemia, sepsis, cirrhosis, arterial venous malformations, or dialysis fistula placement) have a low systemic vascular resistance that showcases a sharp decline in the arterial waveform. Conversely those with a high systemic vascular resistance present with a rather shallow downstroke of the arterial waveform.

Location of catheter placement greatly affects waveform characteristics. Canalization for the continuous monitoring of blood pressure usually takes place in the radial, brachial, or femoral artery. Peak systolic pressure is dependent on the changes in impedance across the vascular tree. Because compliance of the central arterial circulation decreases as blood moves more distantly through the divisions of the arterial tree, distal amplifications in blood pressure measurement occur further away from the aortic root. As a result, there is an increase in systolic blood pressure and a simultaneous decrease in diastolic blood pressure with little to no net effect on MAP in more distal vessels. A latent (or absent) dicrotic notch is seen the further a catheter is placed away from the aortic valve.
Perhaps the most coveted datum obtained from invasive measurement of arterial blood pressure is the MAP. Defined as the average blood pressure during a single cardiac cycle, MAP is a surrogate marker of organ perfusion pressure and greatly influences the autoregulation of blood flow. MAP incorporates principles of cardiac output, systemic vascular resistance, and venous return and is therefore a “holistic” marker of functional cardiovascular health. In the invasive measurement of blood pressure with an arterial catheter, MAP is the geometric mean area under the blood pressure waveform, while in noninvasive blood pressure cuffs it is mathematically obtained from the measured systolic and diastolic pressures. MAP targets are generally recommended by most professional guidelines to direct resuscitative efforts (particularly since they are often resistant to common bedside monitoring errors such as transducer dampening). That said, definitive MAP goals are elusive and vary across different guidelines. Studies comparing MAP targets from 65 to 85 mm Hg show no clear mortality differences in the general population. Paradoxically, targeting higher MAP goals is associated with increasing vasopressor use and the development of untoward cardiovascular effects.
Pulse pressure is another important hemodynamic parameter of arterial pressure obtained from invasive arterial monitoring. It is the difference between systolic and diastolic pressure and represents a pulsatile component of blood pressure (as opposed to the static “point in time” variables represented by MAP). Pulse pressure is proportional to the stroke volume and inversely proportional to aortic compliance. The clinical application of this phenomenon is appreciated when the presence of a narrow pulse pressure indicates a decreased stroke volume (as is the case in cardiogenic shock, aortic stenosis, and various forms of obstructive shock) or a widened pulse pressure that represents an increased aortic compliance or stroke volume (as in hyperdynamic sepsis, anaphylaxis, and thyrotoxicosis).
Monitoring of Cardiac Output With the Pulmonary Artery Catheter
Despite the abundance of data generated from invasive arterial monitoring, blood pressure is, at best, an acceptable surrogate marker for cardiac output. Cardiac output is the major determinant of oxygen delivery and a contributor to end-organ perfusion pressure. The direct monitoring of cardiac output, however, varies in its method of measurement, invasiveness, reliability, and user dependency.
There is no greater gold standard for the assessment of cardiac output than the invasive placement of the pulmonary artery catheter (PAC). Accurate placement of a PAC offers a package of data including intracardiac chamber pressures, the ability to directly assess cardiac output, and mixed venous oxygen saturation. Once ubiquitous, PAC use in critical care units decreased precipitously with publications indicating a lack of clear benefit in hospital outcomes. The introduction of noninvasive methods to measure cardiac output further reduced rates of PAC placement. As a result there is now a potential knowledge deficit in the interpretation of PAC waveforms, which may lead to improper hemodynamic profiling.
As described in Chapter 2 , the PAC varies in length (60–110 cm) and caliber (4–7 French). Cardiac output is measured by the thermodilution method based on the Steward-Hamilton equation or the Fick (direct or indirect) method based on the arteriovenous concentration difference of oxygen (see Chapter 3 ). Choice of PAC placement location in the catheterization laboratory is usually straightforward and dictated by clinician preference and patient clinical needs. The same holds true for the location of PAC placement in the ICU, but selection is also complicated by a lack of readily available fluoroscopic assistance and a long-term need for clinical monitoring. In the ICU setting, the PAC is commonly inserted into the internal jugular vein (potentially leading to inadvertent carotid arterial puncture), subclavian vein (technically more challenging and potentially causing pneumothorax), or femoral vein (associated with higher infection risk and difficulties with daily patient care). PAC use increases the risk of serious complications in the intensive care patient population such as thrombosis, arrhythmias, infections, and difficulties with insertion.
PAC placement is indicated to distinguish cardiogenic from noncardiogenic shock when the clinical examination or noninvasive diagnostic methods are unclear. As the delivery of intravenous fluids is not without its own complications, information obtained from a PAC can also assist with establishing volume resuscitation goals. PAC placement is also indicated to assist with the diagnosis and guidance of therapeutic intervention in patients with advanced heart failure, pulmonary hypertension, complications of acute myocardial infarction, or mechanical circulatory support.
Accurate hemodynamic profile analysis is essential to diagnose different subsets of shock. The derived variables from PAC can assess in describing cardiac function, vasomotor tone, and pulmonary vasomotor tone, in addition to oxygen delivery and metabolism. Consensus guidelines recommend the implementation of less invasive modalities such as echocardiographic assessment before using a PAC and obtaining a solid understanding of patient hemodynamic physiology, pathophysiology, and profiling for adequate interpretation and intervention at the bedside once placement is pursued.
Monitoring of Central Venous Pressure
Measurement of central venous pressure (CVP) may serve as a surrogate marker for cardiac output, and its use is considered when PAC placement is not indicated or unavailable. Transduced off the pressure tracings generated from a central venous line well-placed in the vena caval system, CVP is often described as a static hemodynamic parameter of right atrial pressure ( Fig. 4.2 ). Appropriate CVP measurement is challenging in a vasodilated and volume underloaded (i.e., septic) patient as respiration can cause violent swings in intrathoracic pressure that are often more dramatic with the use of positive-pressure mechanical ventilation. By standardized consensus, CVP measurements are obtained at the end of expiration to avoid the transmural influence of pleural pressure on CVP values.
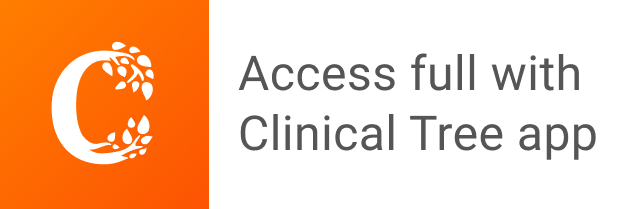