Basic Cardiac Electrophysiology
The aim of this chapter is to cover the main aspects of basic cardiac electrophysiology, developed in a review fashion for the Cardiovascular Medicine Board Examination. The information is organized as follows:
1. Basic action potential (AP) and ion channel implications
2. Electrical activity coupling mechanisms
3. Conduction system anatomy
4. Local electrophysiology characteristics of various conduction system components
MEMBRANE ACTION POTENTIAL
The initiation of the cell membrane AP is the first event in a process that ends with a cardiac contraction. Grossly, myocardial cells can be divided into those dependent on sodium ions (Na+) or calcium ions (Ca2+) to drive AP depolarization.
Sodium-Dependent Cells
Each AP starts with net movement of ions across the cell membrane. In a steady state, the membrane is polarized near -90 mV. The transmembrane ionic current is the result of the balance between many inward and outward ionic currents. The sodium channel is voltage sensitive. This means that the probability of the channel being open for transport of the sodium ion increases with increase of transmembrane voltage (toward zero). When a cell receives depolarizing current, sodium channels open and increase the inward current. When the inward current exceeds the total outward current, a rapid opening of sodium channels occurs that overwhelms any outward current, resulting in the rapid upstroke portion of the AP termed Phase 0 (Figs. 5.1 and 5.2).
FIGURE 5.1 Action potential (sodium channel tissue). AP model of a sodium tissue. Numbers 0, 1, 2, 3, 4 delineate the different phases of the AP.
FIGURE 5.2 Main ion channel activities in AP phases. Na, sodium; K, potassium; Cl, chloride; Ca, calcium.
Sodium channels are characterized by a protein that works as a voltage-gated system. The active portion of this channel is the α subunit, which consists of a 2,000–amino acid glycoprotein. Properties of these channels include the following:
Selective permeation
Gating (activation and inactivation)
Drug binding (local anesthetics)
Susceptibility to many different neurotoxins
Levels of Na+ Channel Activity Regulation
1. Transcriptional regulation of Na+ channel proteins is a mechanism to control Na+ channel expression at a genomic level. This pattern of regulation can be influenced by feedback originating in the tissue electrical activity. The exact mechanism of this gene regulation remains incompletely understood.
2. Phosphorylation/dephosphorylation of the α subunit
3. Glycosylation. The regulation mechanism affects all channel subunits.
Abnormalities of the sodium channel can result in both the long QT syndrome and the Brugada syndrome.
Phase 1 (see Fig. 5.2) starts with the opening of a rapid outward potassium (K+) current called Ito. This determines a fast early repolarization with a prominent notch shape that approximates the membrane potential to 0 mV. These channels are characterized by outward movement of K+ ions, which constitutes the principal source of membrane repolarization early during the AP. The channels inactivate soon after activation, although not as rapidly as the sodium current does. A dynamic interaction of four α subunits and an apparatus composed of a cytoskeleton and signaling complexes mainly form the K+ pore. During the AP, these K+ channels activate in response to membrane depolarization and inactivate in a timed manner. The channels are regulated by:
Angiotensin II, which reduces Ito fast velocity
α-Adrenergic stimulation, which reduces Ito fast velocity
Hyperthyroidism, which increases Ito current density
Aldosterone, which mediates a receptor-specific downregulation of Ito
In human pathophysiology, these channels provide an early repolarization current that can drive the transmembrane voltage toward resting membrane potential when the sodium current is dysfunctional. Thus, in Brugada syndrome, in which there is an abnormality in the sodium current that results in depressed sodium conductance, the Ito current may cause full repolarization in a portion of the myocardium early during the AP, resulting in a large voltage gradient between the repolarized part and parts that have more normal AP. Such gradients have been demonstrated in isolated tissue preparations to be capable of initiating reentrant wavefronts. These reentrant wavefronts can initiate polymorphic ventricular tachycardia or ventricular fibrillation.
The next portion of the AP, termed Phase 2 or the plateau phase (see Fig. 5.2), is the result of the balance of two different ion currents. During Phase 0, at the level of -40 mV, Ca2+ channels open, creating an inward Ca2+ current. This current, acting as an antagonist to the outward K+ current, exerts its action by stabilizing transmembrane potential during the plateau phase. This phase concludes as the Ca2+ current declines by inactivation of L-type Ca2+ channels. These channels are also the critical initiators of cardiac excitation–contraction coupling through the initial increase in intracellular Ca2+, which triggers the release of Ca2+ from the sarcoplasmic reticulum, which in turn provides a contraction signal to the cellular contractile elements. At a level of -40 mV of membrane potential, these channels rapidly activate, reaching a peak in approximately 2 to 7 milliseconds. Inactivation of the channel depends on time, membrane potential, and Ca2+ concentration.
Phase 3 (see Fig. 5.2), the repolarization phase, is dominated by the outward current of K+ through the so-called “delayed rectifier” K+ channels, which are responsible for the return of the cell membrane to its resting polarized state. Two types of delayed rectifiers are important in the repolarization of human ventricular myocardium, a rapidly activating IKr and a more slowly activating IKs that peaks late in the AP, during Phase 3. Abnormalities in either of these two types of delayed rectifier K+ channels can cause the long-QT syndrome.
Phase 4 (see Fig. 5.2) constitutes a stable polarized membrane. This stabilization of membrane AP after the descending Phase 3 is achieved mainly by the action of the voltage-regulated inward rectifiers (IK1). These channels behave differently than the delayed rectifiers, which open in response to depolarization. The inward rectifier K+ channels are opened at near-resting membrane potential, stabilizing the resting membrane potential near the K+ equilibrium potential, but close in response to depolarization, facilitating the AP, hence the description of “inward rectifying.”
Myocardial Tissues That Have Calcium-Dependent AP versus Tissues with Sodium Channels
The main differences between these two types of myocardial tissue can be found in Phases 4 and 0 of the AP. Calciumdependent tissues are the principal cellular component of the specialized conduction system and the sinus node. These cells have the ability to generate a spontaneous AP based on the differential characteristic of Phase 4. This difference is produced by ion currents that affect Na+ and K+ concentrations, called If, which activate at membrane potentials below -40 mV, and the K rectifier currents. These currents confer an unstable electrical property, causing these cells to develop spontaneous diastolic depolarization and automatic onset of APs in a rhythmic fashion. Once spontaneous diastolic activity raises the membrane potential to a value of -40 mV, opening of the slow Ca2+ channels results in an inward Ca2+ current (L-type Ca) that generates the slow AP upstroke (Phase 0). Na+ channels possess a small, if any, role in the AP generation in these particular cells (Fig. 5.3).
FIGURE 5.3 Action potential (calcium channel tissue). AP model of a calcium tissue. Numbers 0, 1, 2, 3, 4 delineate the different phases of the AP.
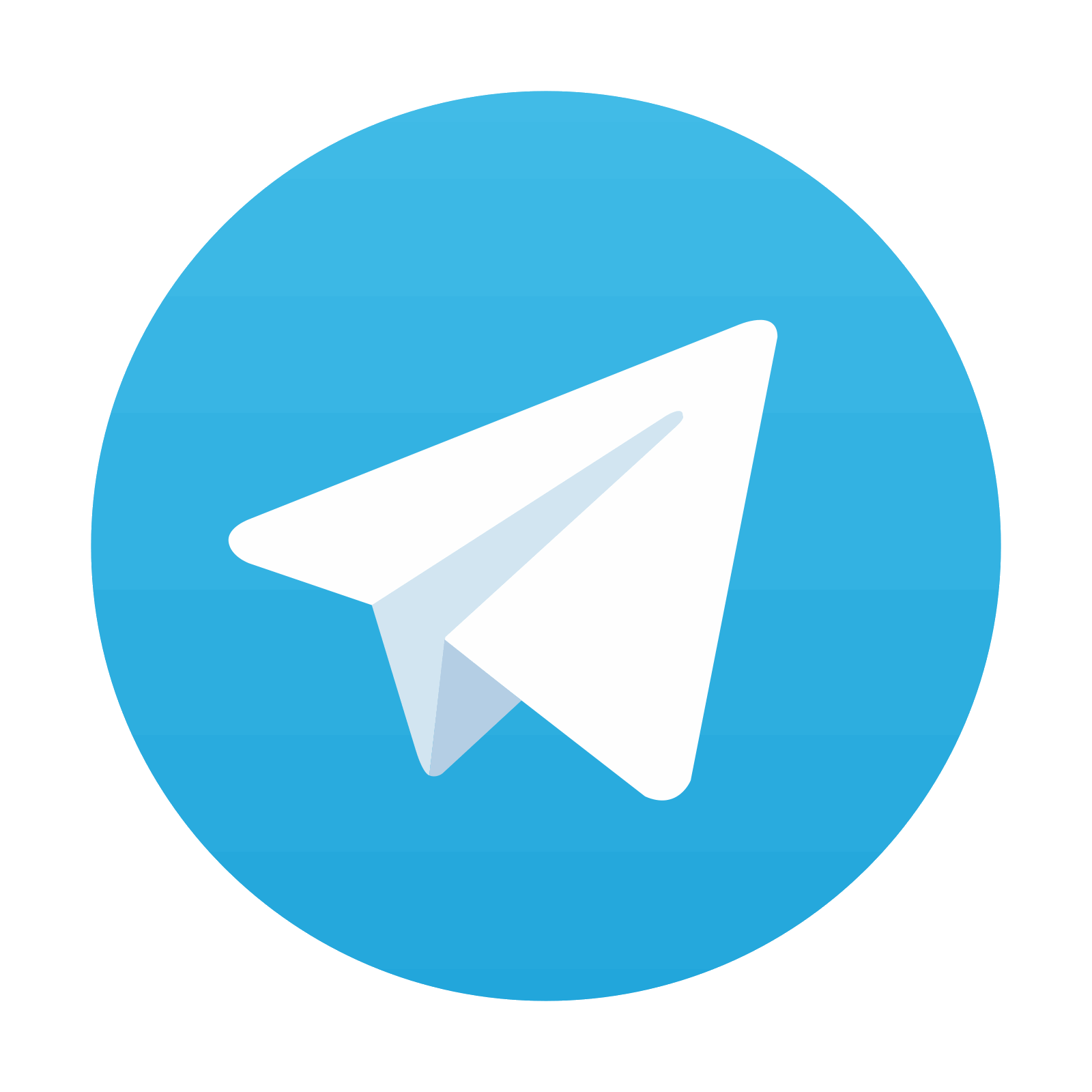