Atherosclerotic Plaque Imaging: MRI
David Rosenbaum
Venkatesh Mani
Antoine Millon
Zahi A. Fayad
ATHEROSCLEROTIC PLAQUES
Atherosclerotic plaques are manifestations of both arterial aging and atherosclerotic process. They occur in the aorta, carotid, and coronary and peripheral arteries, and they are the primary cause of heart disease and stroke thus accounting for 50% of all deaths in Western societies. The initial mechanism of plaque formation is endothelial dysfunction due to systemic (hypertension, diabetes) and/or local (shear stress, turbulences) conditions. It explains the preferential localization of plaques in bifurcations.
The main components of atherosclerotic plaque are: (a) Fibrous elements such as connective tissue, extracellular matrix (including collagen), proteoglycans, and fibronectin elastic fibers; (b) lipids such as crystalline cholesterol, cholesteryl esters, and phospholipids; and (c) inflammatory cells such as monocyte-derived macrophages, T-lymphocytes, and smooth muscle cells (1).
The occurrence of these components in varying proportions in different plaques gives rise to a wide and heterogeneous spectrum of lesions (2,3 and 4). Plaques are mainly developed within the arterial intima but the rearrangement of the wall can also affect the media and the adventitia with, for example, development of neovascularization (5,6). Plaque formation is a very long process that initiates early in life. The natural history of plaques is growth over dozens of years under the influence of local and systemic accelerating factors such as smoking, dyslipidemia, diabetes, hypertension.
There are two main types of complications of atherosclerotic plaques:
Lumen stenosis
The arterial narrowing leads to distal chronic ischemia which first occurs in situations when an increase in O2 intake is needed. This is the mechanism of chronic manifestations of cardiovascular disease such as lower limb claudication and angina pectoris. It has been demonstrated that symptoms only occur after a >60% stenosis is reached (7,8).
Sudden rupture and acute events
Plaque rupture or erosion leads to a sudden thrombus formation and the complete vessel obstruction with possible distal embolization of thrombotic material. This is the mechanism of acute events such as stroke, transient ischemic attack (TIA), or acute coronary syndromes (ACS) (9). Plaque rupture is due to plaque destabilization which has been closely related to its composition, especially with the presence of a lipid core and intraplaque hemorrhage (10,11).
Therefore, within all plaques, a subcategory of vulnerable/high-risk plaques has been defined but the natural history of plaques differs between the localizations of atherosclerosis leading to different types of vulnerable/high-risk plaques. Prone to rupture atherosclerotic coronary artery plaques, so-called “vulnerable” plaques, tend to have a thin (-65 to 150 mm) fibrous cap (FC) and a large lipid core. ACS often results from the rupture of modestly stenotic plaques. Different lesion
types depend in part on stage or phase of atherosclerosis progression. The coronary “vulnerable” types IV and Va lesions and the “complicated” type VI lesion are the most relevant to ACS according to the criteria of the American Heart Association Committee on Vascular Lesions. Those types IV and Va lesions, although not necessarily stenotic at angiography, frequently have increased density of resident macrophages that release proteolytic enzymes such as metalloproteinases (12) that degrade the FC and increase the risk of plaque rupture. Type IV lesions consist of extracellular lipid intermixed with fibrous tissue that is covered by a FC, whereas type Va lesions possess a predominantly extracellular lipid core covered by a thin FC. The lipid core is made highly thrombogenic by the presence of tissue factor (13). The type VI lesion is formed after disruption of type IV or Va lesions and results in ACS, consists of an occlusive thrombus. The majority of atherosclerotic lesions in the coronary arteries are minimally stenosed (14) but these relatively nonstenotic plaques with large lipid cores are actually vulnerable and at high risk for rupture and thrombosis. The plaque caps are often thinnest at the shoulder regions, where macrophages (15) and mast cells (16) accumulate, and disruption is a frequent occurrence (12). In contrast, the most severely stenotic plaques at angiography, which have a high content of smooth muscle cells and collagen, and little lipid, are less susceptible to rupture.
types depend in part on stage or phase of atherosclerosis progression. The coronary “vulnerable” types IV and Va lesions and the “complicated” type VI lesion are the most relevant to ACS according to the criteria of the American Heart Association Committee on Vascular Lesions. Those types IV and Va lesions, although not necessarily stenotic at angiography, frequently have increased density of resident macrophages that release proteolytic enzymes such as metalloproteinases (12) that degrade the FC and increase the risk of plaque rupture. Type IV lesions consist of extracellular lipid intermixed with fibrous tissue that is covered by a FC, whereas type Va lesions possess a predominantly extracellular lipid core covered by a thin FC. The lipid core is made highly thrombogenic by the presence of tissue factor (13). The type VI lesion is formed after disruption of type IV or Va lesions and results in ACS, consists of an occlusive thrombus. The majority of atherosclerotic lesions in the coronary arteries are minimally stenosed (14) but these relatively nonstenotic plaques with large lipid cores are actually vulnerable and at high risk for rupture and thrombosis. The plaque caps are often thinnest at the shoulder regions, where macrophages (15) and mast cells (16) accumulate, and disruption is a frequent occurrence (12). In contrast, the most severely stenotic plaques at angiography, which have a high content of smooth muscle cells and collagen, and little lipid, are less susceptible to rupture.
In contrast to vulnerable plaques in the coronary arteries, high-risk plaques in the carotid arteries are more often severely stenotic. The term high-risk, rather than the classic term vulnerable, should be preferred because vulnerable implies the presence of a lipid-rich core. Indeed, high-risk carotid plaques are not necessarily lipid rich but rather heterogeneous, and are very rich in fibrous tissue. These plaques often become symptomatic due to an intramural hematoma or dissection that likely develops secondary to the impact of blood during systole against a resistant area of stenosis (17). Since the carotid arteries are superficial and not subject to significant motion, they are much easier to image than the coronary arteries, and therefore, they are the most studied arteries with MRI (18). In the thoracic aorta, atherosclerosis is a significant marker of associated coronary disease (19,20). In fact, parameters such as aortic wall thickness, luminal irregularities, and plaque composition are strong predictors of future vascular events (21). These plaques correspond to noncalcified, frequently lipid-laden plaques (American Heart Association types IV and Va) as described in the coronary arteries. They have been found to be associated with a significantly increased risk for all vascular events (stroke, myocardial infarction, peripheral embolism, and cardiovascular death) in patients who had noncalcified aortic plaques with a thickness of more than 4 mm in the French Aortic Plaque Study (FAPS) (17,21).
In summary, the useful information that could be provided by atherosclerotic plaque imaging with MRI are:
Anatomic imaging
Plaque geometry: Size, localization, and percent of stenosis.
Plaque internal composition
Functional imaging
Assessment of plaque internal biologic mechanisms
EVOLUTION over time with monitoring of potential therapeutic effects of prescribed medications.
MRI TECHNIQUES
In the past, the degree of arterial stenosis was considered the main determinant factor in determining the need to treat a patient. This notion has, however, in recent years evolved to include several other factors that are considered potentially important markers for future cerebrovascular events. Some of these factors include plaque composition, size of the FC, intraplaque hemorrhage, plaque ulceration and plaque location, and the size of a lipid-rich necrotic core. This has been facilitated primarily by the development of dark blood MR imaging techniques that offer high-resolution direct visualization of plaque components.
MAGNETIC RESONANCE ANGIOGRAPHY
The most commonly clinically used MR imaging for visualizing atherosclerosis is the magnetic resonance angiography (MRA) approach. This is achieved using both noncontrastenhanced imaging (time-of-flight (TOF) angiography) or contrast-enhanced angiography using Gd-chelates. MRA does not require any sophisticated postprocessing approach to get rid of calcium-related artifacts, reducing image interpretation time with a simple and fast luminographic display of the arterial anatomy, superimposable to that of DSA.
PLAQUE MORPHOMETRICS
The direct visualization of atherosclerotic plaques should enhance our understanding of the natural history of this disease. Several invasive imaging techniques, such as x-ray angiography, intravascular ultrasonography, angioscopy, and optical coherence tomography, in addition to noninvasive imaging techniques, such as surface B-mode ultrasonography and ultrafast computed tomography, can be used to assess atherosclerotic vessels. Most of the standard techniques identify the luminal diameter or stenosis, wall thickness, and plaque volume. However, none of these imaging methods can completely characterize the composition of the atherosclerotic plaque, and therefore, have inadequate sensitivity and specificity to identify vulnerable or high-risk plaques (22).
High-resolution MR has emerged as the potential leading noninvasive imaging modality for characterizing atherosclerotic plaque in vivo. With improvements in imaging technology, the ability of magnetic resonance (MR) to delineate these vessels has significantly improved (23,24,25,26,27,28 and 29). MR differentiates plaque components on the basis of biophysical and biochemical parameters, such as chemical composition and concentration, water content, physical state, molecular motion, and diffusion. MR provides a method of imaging without the need for ionizing radiation that can be repeated sequentially over time. Dedicated pulse sequences have become more readily available for time-efficient multislice imaging. Over time, a diverse array of black blood MRI techniques (30,31) has developed into practical tools for the arterial imaging and evaluation of atherosclerosis.
In black blood MRI, images are commonly acquired using a rapid acquisition with relaxation enhancement (RARE) sequence with double inversion recovery (DIR) pulses (30) to provide good contrast between the lumen and vessel wall. DIR modules consist of two 180-degree radiofrequency (RF) pulses. Magnetization in the whole volume is inverted by the first nonselective RF pulse, while magnetization in the slab of interest is subsequently restored by the second selective RF pulse. The application of these two preparatory RF pulses causes spins outside the slab of interest to be inverted, with no effect on spins within the slab. Image acquisition begins following a time delay (TI) necessary for magnetization of inverted blood flowing into the imaging slice to reach null point (30). DIR techniques have been modified for multislice black blood imaging (23,24,27,32). The use of black blood MRI to directly image atherosclerotic plaques provides the unique opportunity of measuring plaque and wall changes due to atherosclerotic disease with high accuracy, taking into account the intrinsic variations of the diseased arterial wall. One technique for multislice black blood imaging is the rapid extended coverage (REX) (24) method. This method allows for imaging up to 20 times faster than the conventionally used single-slice, black blood DIR technique. More recently, these two-dimensional (2D) approaches have given way to three-dimensional (3D) dark blood imaging techniques. It is an increasingly common imaging approach employed in multiple vascular beds including the carotid (33), aorta (34) and femoral (35) arteries in an attempt to evaluate systemic disease burden instead of focusing on specific lesions. In such instances, 3D black blood approaches can subsequently be used as a measure to evaluate changes in burden in response to treatment and/or evaluate progression or regression of disease over time (36). Due to a higher SNR and decreased susceptibility of volume averaging artifacts, 3D approaches are often preferred to the conventional 2D approaches. The ability to obtain isotropic voxels thereby enabling multiplanar reformatting of images is yet another benefit of 3D approaches. The most promising 3D black blood turbo spin-echo (TSE) sequence approach currently being investigated is the sampling perfection with applicationoptimized contrast using different flip angle evolution (SPACE) approach (37). Other 3D approaches include the use of a steady-state free precession (SSFP) approach combined with a diffusion preparation for nulling blood signal (35) and the MSDE approach with a TSE acquisition kernel. New automated segmentation approaches for plaques have also been proposed in different vascular territories such as carotids (38) and femoral arteries (39).
PLAQUE CHARACTERIZATION
The main advantage of MRI over other noninvasive imaging modalities is its ability to characterize plaque components and help determine constituents that may be vulnerable to rupture. The characterization of atherosclerotic plaque by MR is based on the signal intensities and morphologic appearance of plaque on T1-weighted, proton-density-weighted, and T2weighted images, as previously validated (18,40,41). The fibrous tissues of plaque, consisting of extracellular matrix elaborated by smooth muscle cells, are associated with a short T1. This T1-shortening (increased signal intensity on T1-weighted images) is the consequence of specific interactions between protein and water (42). Plaque lipids consist primarily of unesterified cholesterol and cholesteryl esters and are associated with a short T2 (41). The short T2 (decreased signal intensity of T2-weighted images) of the lipid components is in part a consequence of the micellar structure of lipoproteins, their denaturation by oxidation, or an exchange between cholesteryl esters and water molecules (both from the fatty chain or the cholesterol ring), with a further interchange between free and bound water (43,44) (Fig. 27.1). Perivascular fat, mainly composed of triglycerides, differs in appearance on MR images from atherosclerotic plaque lipids (45). The signal intensities of the calcified regions of plaque, which consist primarily of calcium hydroxyapatite, are low on MR images
because of their low proton density and diffusion-mediated susceptibility effects (46).
because of their low proton density and diffusion-mediated susceptibility effects (46).
The MR appearance and evolution of thrombus or hemorrhage in the central nervous system (47), pelvis (48), and aorta (49,50) has been studied. The identification of hemorrhage with MRI depends on the structure of hemoglobin and its oxidation state. At various stages, blood clots contain different products (i.e., oxyhemoglobin, deoxyhemoglobin, methemoglobin, hemosiderin/ferritin). Each of these has a set of specific MR relaxation properties (T1 and T2) that produce different signal intensities (51,52,53,54 and 55). Blood shortens the T1 and T2 of water. Presumably, T1-shortening is caused by the formation of methemoglobin (which is paramagnetic) from hemoglobin, whereas T2 shortening is caused by magnetic susceptibility. In this regard, ferritin- or hemosiderinrich mature thrombus is associated with marked signal loss on T2-weighted images.
As mentioned previously, most in vivo studies of MR imaging and characterization of plaque have been performed in a multicontrast approach (i.e., T1-weighting, protondensity-weighting, and T2-weighting) with high-resolution black blood spin-echo and fast spin-echo (FSE) MR sequences. As the name implies, the signal from flowing blood is rendered black by the use of preparatory pulses (e.g., RF spatial saturation or inversion recovery pulses) for better visualization of the adjacent vessel wall. Hatsukami et al. (56) introduced the use of bright blood imaging (i.e., 3D fast TOF imaging) to visualize FC thickness and morphologic integrity. This sequence provides enhancement of the signal from flowing blood and a mixture of T1-weighting and proton-density contrast-weighting, which highlights the FC. Multicontrast MRI of human carotid plaque had high sensitivity and specificity for identification of unstable FCs and may enable prospective identification of high-risk carotid plaques (57).
In addition, MR direct thrombus imaging using a T1weighted turbo field-echo sequence, which improves detection of methemoglobin, enabled accurate identification of complicated carotid lesions with thrombus in symptomatic patients that underwent imaging and subsequent endarterectomy (58,59).
Measurement of carotid wall volume and maximum area using 3D contrast-enhanced MR has also been performed (60). MRA and high-resolution black blood imaging of the vessel wall can be combined. MRA demonstrates the severity of stenotic lesions and their spatial distribution, whereas the high-resolution black blood wall characterization technique may show the composition of plaques and facilitate risk stratification and selection of treatment modality. Spatial resolution has recently been improved (≤250 µm) with the design of new phased-array coils (61,62) tailored for carotid arteries and new imaging sequences, such as long echo train FSE imaging with “velocity-selective” flow suppression and DIR preparatory pulses (black blood imaging) (60,61,63).
Following surgery, the carotid plaque specimens were again imaged ex vivo with high-resolution MRI and revealed good correlation between in vivo and ex vivo measurements of maximum wall area, minimum lumen area, and wall volume. High-resolution MRI also had good sensitivity and specificity for determining the type of carotid lesion based on the American Heart Association classification system (64,65 and 66).
MRI has been used to identify intraplaque hemorrhage (67) and also differentiate intraplaque and juxtaluminal hemorrhage/thrombus in advanced human carotid atherosclerosis (68,69). The use of gadolinium-based contrast agents has enabled improved characterization of carotid plaque by not only improving visualization of the arterial lumen but also by differential contrast enhancement of various plaque components. For example, Yuan et al. (70,71) demonstrated an 80% enhancement of fibrous tissue while there was only a 29% enhancement of the necrotic core when comparing postcontrast-enhanced with noncontrast-enhanced highresolution MRI. MRI has also been used to study differences in carotid plaque composition between different ethnic and racial groups (72).
DYNAMIC CONTRAST-ENHANCED MRI
The formation of neoangiogenic vessels has been associated with the hypoxic conditions in the thickened intimal layer (>500 µm), and recent observations suggest that neovascularization may promote plaque inflammation and progression already at an early stage of plaque formation by facilitating extravasation of inflammatory cells. Plaque growth has also been intimately associated with intraplaque hemorrhage of the fragile neovasculature. Dynamic contrast enhancement (DCE) MRI is a well-validated technique examining the vascularity of tumors. In the past few years, this technique has been adapted for use in atherosclerotic plaque neovascularization in both animal models (73,74 and 75) and in humans (76). DCE-MRI uses repeated MRI acquisitions of the same imaging slice/volume serially with high temporal resolution after the injection of a Gd-based contrast agent. DCE-MRI has demonstrated the ability to differentiate fibrous tissue (enhancement ˜80%) from lipid core (enhancement -30%) by virtue of the neovessel density in fibrous tissue. It has also been shown to correlate well with neovessel density on histology (77,78) (Fig. 27.2). Both model-based and modelfree approaches to analyzing DCE images have been proposed and implemented. Appropriate modeling of the signal intensity time course in the plaque provides information on contrast agent plaque uptake kinetics and estimations of the amount of neovascularization and vessel permeability (79).
TARGETED MOLECULAR CONTRAST AGENTS IMAGING
The ability to target specific molecules of plaques may greatly enhance detection and characterization of atherosclerotic and atherothrombotic lesions using MRI (80). Contrast agents linked to antibodies (81,82,83 and 84) or peptides (85,86) that target specific plaque components or molecules that localize to specific regions of atherosclerotic plaque (25,87,88 and 89) are examples of strategies that have been employed to image atherosclerosis with MRI.
The ability to target mononuclear cells such as monocytes, macrophages, and foam cells is an attractive means of identifying atherosclerosis since these cells have been shown to play a pivotal role in the progression of atherosclerosis to symptomatic disease (90,91). While current research is underway to target macrophages with gadolinium-based contrast agents that target the macrophage scavenger receptor,
macrophages have only been imaged with iron oxide compounds (USPIOs, SPIOs) that are removed from the circulation by macrophages and other cells of the reticuloendothelial system (87,88 and 89). In a study by Kooi et al. (88) on 11 symptomatic patients scheduled for carotid endarterectomy, 75% of ruptured or rupture-prone lesions demonstrated uptake of USPIOs compared with only 7% of stable lesions and a decrease in signal intensity of 24% on T2*.
macrophages have only been imaged with iron oxide compounds (USPIOs, SPIOs) that are removed from the circulation by macrophages and other cells of the reticuloendothelial system (87,88 and 89). In a study by Kooi et al. (88) on 11 symptomatic patients scheduled for carotid endarterectomy, 75% of ruptured or rupture-prone lesions demonstrated uptake of USPIOs compared with only 7% of stable lesions and a decrease in signal intensity of 24% on T2*.
Neovascularization has been shown to play an important role in atherosclerosis and the integrin αvβ3 has been targeted to identify regions in the vessel wall undergoing neovascularization (82,83). Winter et al. (84,92) recently demonstrated in a rabbit model of atherosclerosis that regions of neovascularization in plaque had a 47% increase in signal intensity following treatment with αvβ3-targeted nanoparticles.
Additional targets of interest for imaging of atherosclerotic plaque with molecular specific MR contrast agents are oxidized low-density lipoprotein (oxLDL), tissue factor, endothelial integrins, matrix metalloproteinases, and extracellular matrix proteins such as tenascin-C.
While targeted nuclear imaging through the use of antibodies specific to oxLDL has been demonstrated promises at detecting atherosclerosis (93), estimating plaque volume (94), and following progression/regression of atherosclerosis (95). Recently, studies evaluating oxLDL as a target for molecular MRI of atherosclerosis have been performed (96,97). In addition, molecules such as tissue factor (85) and endothelial integrins (98,99 and 100) such as E-selectin, P-selectin, intracellular adhesion molecule-1, or vascular cell adhesion molecule-1 have been targeted with antibodies linked to echogenic contrast agents.
While these agents utilize echocardiography or nuclear imaging, the echogenic or nuclear contrast agent could easily be replaced by linking an MR contrast agent to the monoclonal antibody to target the molecule of interest. However, the identification of molecules found only in atherosclerotic plaque will ultimately enable improved detection of atherosclerotic plaque assuming that the target is expressed in adequate quantity for detection by molecular MRI.
Gadofluorine M is a lipophilic, macrocyclic (1,528 Da), water-soluble, gadolinium chelate complex (Gd-DO3Aderivative) with a perfluorinated side chain. Sirol et al. (25) and Barkhausen et al. (101) demonstrated that Gadofluorine M enhanced aortic wall imaging in Watanabe heritable hyperlipidemic rabbits but did not enhance the aorta of control rabbits. Gadofluorine M increased signal intensity by 164% at 1-hour postcontrast and increased signal intensity 207% at 24-hour postcontrast.
The ability to identify components of thrombus with molecular MRI may enable enhanced detection and characterization of both luminal thrombus and components of thrombus organized in an old atherothrombotic lesion. Therefore, selection of targets pivotal in the coagulation cascade, such as fibrin, factor XIII, integrins on the surface of platelets, and tissue factor, is necessary to identify the areas of old or active thrombus formation. The attachment of MR contrast agents to both monoclonal antibodies or peptide ligands that specifically bind components of thrombus has been performed in animal models (102,103 and 104) and humans (92,105). In addition, thrombus resulting from plaque rupture has been identified using fibrin-specific MR contrast agents in a rabbit carotid crush injury model (106,107). In the 25 arterial thrombi induced by carotid crush injury, Botnar et al. (106) demonstrated a sensitivity and specificity of 100% for in vivo thrombus detection using MRI. Sirol et al. (107) investigated a similar
fibrin-specific MR contrast agent in 12 guinea pigs demonstrating that thrombus signal intensity was increased by over fourfold after intravascular delivery of contrast agent and thrombus was detected in 100% of animals postcontrast compared with 42% identification of thrombus precontrast (107).
fibrin-specific MR contrast agent in 12 guinea pigs demonstrating that thrombus signal intensity was increased by over fourfold after intravascular delivery of contrast agent and thrombus was detected in 100% of animals postcontrast compared with 42% identification of thrombus precontrast (107).
Inflammatory activity in atherosclerotic plaque is also identified as a strong risk factor for plaque rupture and progression and may be used as an imaging target to evaluate interventional therapy. While inflammatory activity is typically assessed in vivo using 18-FDG-PET, some approaches using MRI have also been proposed. Inflammatory activity can be evaluated at the (sub) cellular level using in vivo molecular MRI. Various MRI contrast agents, such as ultra-small particles of iron oxide, micelles, liposomes, low-molecular-weight Gd-chelates, and perfluorocarbon emulsions, have been used for in vivo visualization of various inflammation-related targets, such as macrophages, oxLDL, endothelial cell expression, plaque neovasculature, MMPs, apoptosis, and activated platelets/thrombus (Fig. 27.3).
IMAGING OF PlAQUE BIOMECHANICE
The overarching goal of plaque imaging is to identify plaque features that predict risk of clinical events. Biomechanical features of vulnerable plaques can be exploited to help stratify this plaque risk. Therefore, a single morphologic feature may not truly represent the total vulnerability of the plaque, but it may be a combination of all the biomechanical features of these vulnerable features (108). Several studies have linked biomechanical forces to the pathogenesis of atherosclerotic plaque implying that biomechanical analyses may reveal a predisposition for vulnerable plaque development. Thus plaque biomechanics play a vital role in determining hallmarks of vulnerability (109).
Plaque mechanics using MRI are typically performed using computational models derived from vessel wall morphology. Computational fluid dynamics (CFD) provides an elegant method to derive geometry and flow boundary conditions from MR images (110). By modeling blood flow using patient- and vessel-specific geometry and blood flow conditions consists of many steps. Some of these steps include
image segmentation, geometric reconstruction, mesh generation, determination of boundary conditions, and numerical analysis (111). Alternatively, MRI can also directly characterize arterial flow patterns, as well as measure blood flow velocity using phase-contrast MRI (PC-MRI). The velocity information provided by PC-MRI can be used to compute wall shear stress maps along different arterial segments (112). MRI techniques also exist to directly measure tissue strain. Circumferential strain can be measured by displacement encoding with stimulated echoes (DENSE) agreed and has shown correlations with CINE measurements of changes in lumen circumference in normal carotid arteries. Such strain imaging approaches, however, may not have the required resolution to measure radial strain, another important strain component (113).
image segmentation, geometric reconstruction, mesh generation, determination of boundary conditions, and numerical analysis (111). Alternatively, MRI can also directly characterize arterial flow patterns, as well as measure blood flow velocity using phase-contrast MRI (PC-MRI). The velocity information provided by PC-MRI can be used to compute wall shear stress maps along different arterial segments (112). MRI techniques also exist to directly measure tissue strain. Circumferential strain can be measured by displacement encoding with stimulated echoes (DENSE) agreed and has shown correlations with CINE measurements of changes in lumen circumference in normal carotid arteries. Such strain imaging approaches, however, may not have the required resolution to measure radial strain, another important strain component (113).
APPLICATIONS
MEASUREMENT OF PLAQUE SIZE
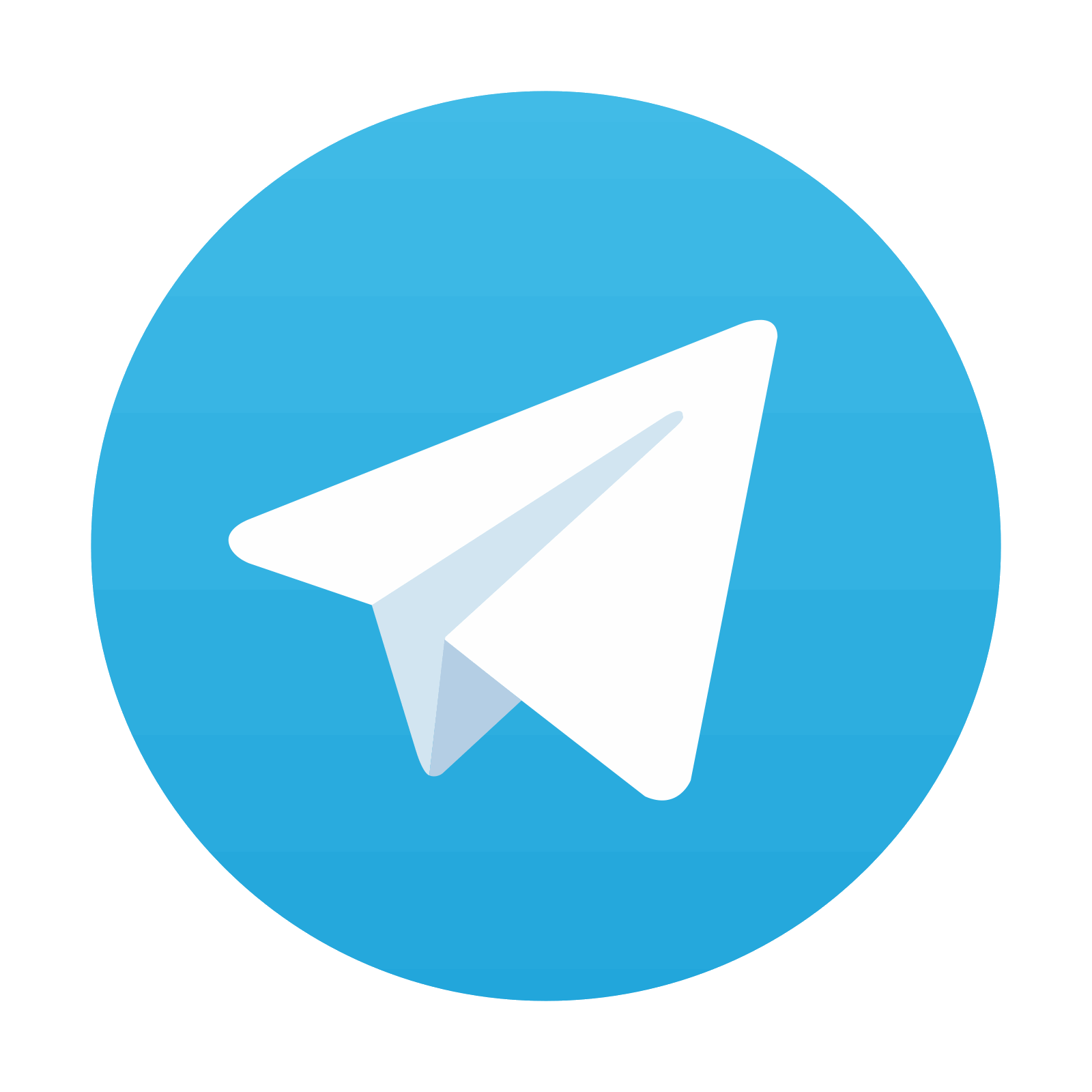
Stay updated, free articles. Join our Telegram channel
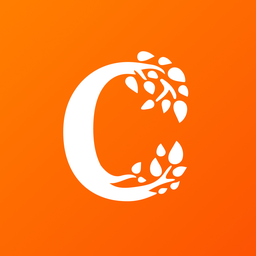
Full access? Get Clinical Tree
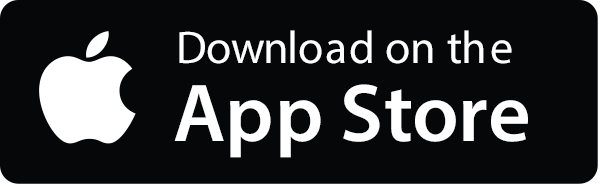
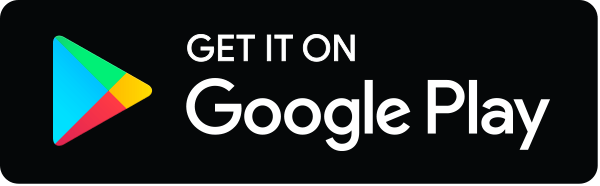