Fig. 15.1
Progression of atherosclerosis with time. a Healthy artery showing the various layers, from the outside these are the adventitia, media and intima. b The first sign of disease is fatty streaks seen in the intima; these usually appear from about the first decade of life. c As the disease progresses there is thickening of the intimal layer; often associated with lipid deposition and the presence of foam cells. d An early plaque is formed consisting of a lipid pool, and there are calcified regions, foam cells and cholesterol clefts. The inner lumen is unaffected due to outward remodelling. Early plaque appear from about the third decade of life. e and f Late plaque is associated with reduction in the lumen diameter; there may be larger regions of calcification. The wall between the lumen and the lipid pool (the cap) is of variable size. In (e) the cap is thick, in (f) the cap is thin—thin cap plaque are at higher risk of rupture. Late plaque appear from about the fourth decade of life

Fig. 15.2
Plaque biology basics. This involves; adhesion of monocytes to the endothelial surface (1), migration into the vessel wall (2), differentiation into macrophages (scavenger cells) (3). Movement of LDL cholesterol between the endothelial cells and into the subendothelial space (4) where they are oxidised and ingested by macrophages (5) forming foam cells (6) which reach a certain size and then rupture depositing their lipids in the vessel wall (7) which attracts more monocytes (8). Migration of vascular smooth muscle cells (smcs) from the media into the intima (9) and smc proliferation under the control of cytokines (10)
All these phenomena (local lumen reduction, spillage of contents and local thrombosis) combine to cause reduced perfusion to the tissues supplied by the artery. If perfusion reduces below a critical amount then there is tissue death. The tissue which is affected may have an alternative supply from a different artery in which case perfusion may be impaired but still sufficient for tissue viability. Often plaque rupture is the event that triggers symptoms in the patient. Plaque rupture in the carotid arteries typically leads to death of brain tissue (stroke). Plaque rupture in the coronary arteries typically leads to death of cardiac tissue (heart attack).
For arteries supplying the legs (aorta, iliac, femoral), the effects of atherosclerosis are often less dramatic. As atherosclerosis progresses then a secondary (collateral) circulation develops which enables blood to bypass the affected artery. The first sign of disease is claudication which is pain in the calf after walking. The distance the patient can walk (the claudication distance) gradually decreases until the patient has pain at rest. Any further deterioration leads to inadequate blood supply which if untreated can lead to tissue death and gangrene.
Treatment of disease is usually associated with re-establishing adequate perfusion, and in some cases surgical removal of dead tissue through amputation. Re-perfusion treatment can involve bypassing the affected artery using a vein taken from the patient or using an artificial graft. The atherosclerotic plaque may be surgically removed if the artery is accessible, such as the carotid artery. The artery may be rebored using catheter techniques under X-ray guidance. Stents may be used in an attempt to stop re-stenosis.
15.2 Biomechanics
Atherosclerosis causes considerable changes to the elastic properties of the vessel wall and to blood flow and pressure which are described in this section.
15.2.1 The Flow-Field in Stenoses
The effect of a stenosis on local blood velocities is illustrated in Fig. 15.3. This is an idealised 70 % stenosis by diameter with steady flow. As the blood flows through the stenosis there is an increase in velocity. This may be understood in that, as the cross-sectional area A decreases, the velocity v increases. The flow rate is the product of the cross-sectional area and the mean velocity



Fig. 15.3
Schematic of flow characteristics in an idealised 70 % stenosis in which turbulence is ignored. Velocity increases within the stenosis and a jet is formed. In the immediate post-stenosis region there is boundary separation with an area of recirculating flow. Fully developed flow is re-established some diameters downstream

(15.1)
Flow rate is constant at all cross sections along the vessel so that a decrease in A must lead to an increase in v. It might be thought that the maximum velocity would be located at the point of minimum lumen however this is not the case. The convergence of the flow streamlines which occur in the immediate pre-stenotic region continues and the minimum diameter of the flow stream is located just downstream of the minimum lumen; this region is called the ‘vena contracta’. The maximum velocity is located at the vena contracta, not at the point of minimum lumen. Immediately downstream of the stenosis there is a region of flow recirculation. Flow separation occurs and there is a boundary layer separating the two flow regions. The boundary layer reattaches to the vessel wall downstream as shown in Fig. 15.3b
Changes in the velocity profiles are shown in Fig. 15.3b. The velocity profile which is initially parabolic becomes almost flat within the stenosis. In the post-stenotic region the velocity profile has small regions of reverse flow. Due to viscosity, the effect of the stenosis on the flow patterns diminishes with distance. After several diameters downstream the jet dies out and the parabolic velocity profile resumes. In this idealised example the effect of turbulence has not been considered, and the region of recirculation is stable. In real stenoses in arteries the post-stenotic region may be highly unstable with turbulence and detachment of the post-stenotic vortices which are swept downstream. Figure 15.4 shows examples of flow-field data taken in simulated stenoses.


Fig. 15.4
Flow-field data in idealised stenoses. a 50 % stenosis with velocities estimated using CFD with an LES model of turbulence. The jet is seen in red with recirculating flow in blue and turbulence several diameters downstream. b Planar carotid bifurcation model with a 50 % stenosis with velocities estimated using PIV. The jet is seen in red with recirculation of flow in purple and blue. Figure 15.4b reprinted from; Journal of Biomechanics, Vol. 47(1); Kefayati S, Holdsworth DW, Poepping TL; Turbulence intensity measurements using particle image velocimetry in diseased carotid artery models: Effect of stenosis severity, plaque eccentricity, and ulceration; pp. 253–263, Copyright (2014); with permission from Elsevier
The changes which the stenosis makes on the velocity field will cause changes to the wall shear stress patterns (Fig. 15.5). The maximum wall shear stress is located just before the minimum lumen. The post-stenotic region has low wall shear stress values. The peak wall shear stress in the tighter stenosis is 180–200 Pa. These high values of wall shear stress are thought to be responsible for the endothelial stripping which commonly occurs in tight stenoses in vivo. The distribution of wall shear stress is relevant in terms of the growth of atherosclerotic plaque as described in Sect. 15.3.


Fig. 15.5
Wall shear stress in idealised stenosed straight arteries estimated using CFD, with a 30 % stenosis and b 70 % stenosis. In each case the peak wall shear stress occurs just before the point of minimum lumen and there is negative shear stress in the immediate post-stenotic region. WSS images from; Li (2007); reprinted with permission from the author
15.2.2 Pressure Changes Across Stenoses
To investigate the change in pressure as blood flows through a stenosis we will initially consider flow of an idealised fluid in which there is no loss of energy in which case the simplified version of Bernoulli’s equation can be used

where P is pressure, h is height, ρ is density, g is the gravitational constant and v is velocity.

(15.2)
In Eq. 15.2 an increase in velocity is associated with a reduction in pressure, and vice versa. As blood flows through a stenosis there will be an increase in velocity and consequently a reduction in pressure (Fig. 15.6b). The point of maximum velocity and minimum pressure is located at the vena contracta. Downstream from the vena contracta the velocity decreases and so pressure increases, eventually recovering to its pre-stenosis value. This may be explained in terms of energy. The energy of the blood is shared between energy associated with pressure and kinetic energy (associated with movement of the blood). In this simplified example the total energy is constant so a reduction in pressure energy is associated with an increase in kinetic energy and vice versa.


Fig. 15.6
Pressure as a function of axial distance in an artery with a 70 % stenosis (same geometry as Fig. 15.3). a Vessel geometry. b Flow of blood with no viscosity or turbulence (i.e. no energy loses). The pressure falls reaching a minimum value corresponding to the point of minimum lumen (at the vena-contracta); with increasing distance from the stenosis there is increase in pressure with full recovery to the pre-stenotic value. c Flow of blood including energy losses due to viscosity and turbulence. There is some recovery of pressure in the post-stenosis region but overall there is a pressure drop resulting from viscous and turbulent energy losses
In reality there are energy losses due to viscosity and turbulence. This leads overall to a pressure drop as the blood passes through the stenosis as shown in Fig. 15.6c.
Measurements of the pressure drop are widely used in cardiology in the coronary arteries where decisions are made as to which stenosis to treat and whether the effect of treatment has been successful (Sect. 15.5.2).
15.2.3 Flow Rate and Velocity as a Function of Degree of Stenosis
It might be expected that even a small reduction in artery diameter would cause some reduction in flow rate in the artery. However studies on animals with artificial stenosis and flow monitoring probes have shown that the flow is unaffected until the degree of stenosis reaches about 70 % by diameter (91 % by area), (Berguer and Hwang 1974). The value of lumen reduction after which flow decreases is called the ‘critical stenosis’. Figure 15.7 shows the effect of stenosis value on flow rate and blood velocity. The velocity increases from 20 cm.s−1 in an unstenosed artery to a maximum value of 600 cm.s−1 at 84 % stenosis. It will be described in Sect. 15.4, in terms of clinical measurement of the degree of stenosis, that it is velocity which is used as flow rate does not distinguish different degrees of stenosis.


Fig. 15.7
Flow rate and velocity as a function of degree of stenosis. Flow rate is maintained constant up to 70 % by diameter after which flow reduces. The diameter of the artery and downstream arterioles are illustrated. As disease progresses the arterioles compensate by dilating in order to maintain flow constant. At 70 % stenosis the arterioles are fully dilated. Further increase in disease cannot be compensated by further arteriolar dilation and flow reduces. Drawn from data in Spencer and Reid (1979)
This data may be explained using the simple model of the arterial system described in Chap. 5, which is shown in Fig. 15.8. The flow rate is determined by the pressure gradient and by the overall resistance. The overall resistance is a combination of the resistance in the large arteries and in the arterioles. When a stenosis develops, the resistance in the large arteries increases. This is compensated by a reduction of resistance in the arterioles which is caused by dilation of the arterioles. Hence the overall resistance is maintained constant and the flow rate is also maintained constant. The arterioles are fully dilated when the stenosis value is 70 % by diameter (Fig. 15.7). Further increase in the degree of stenosis leads to increase in overall resistance and the flow rate decreases.


Fig. 15.8
Simple model of flow consisting of a heart, an artery and a downstream arterioles. Disease in the artery increases resistance. This is compensated by decrease in resistance of the arterioles
The concept of critical stenosis is useful in understanding the haemodynamic effects of stenosis, however in practice other mechanisms come into play to help preserve perfusion to the organ. The organ may be perfused by several arteries so that flow reduction in one artery does not have a drastic effect on perfusion. In the brain, the Circle of Willis acts to connect the different arteries which supply the brain. If the Circle of Willis is complete, then a stenosis in one artery (e.g. left internal carotid artery) may have a relatively minor impact on perfusion of the brain as the supply of blood can be taken over by other arteries. However if the Circle of Willis has missing connections then this resupply from other arteries is less effective and there will be reduced perfusion to the relevant area of the brain which may lead to a stroke. Atherosclerosis in arteries in the leg leads to reduction in flow rate in the artery. However, downstream (distal) tissues may be supplied by a collateral circulation which bypasses the diseased region. The collateral circulation consists of many small arteries which grow in size as the degree of stenosis in the main artery increases. The severity of symptoms (claudication, gangrene) depends to a large extent on how well the collateral circulation matures. Patients may have complete occlusion of both superficial femoral arteries and be unaware of this as they have very well developed collateral circulations.
15.2.4 Plaque Stiffness and Wall Stress
From a mechanical point of view an atherosclerotic plaque has two main regions; a lipid pool surrounded by a fibrous region (Fig. 15.9). The region of the plaque between the lipid pool and the lumen is called the ‘cap’, and rupture of the cap triggers clinical events such as stroke and heart attack. Plaque rupture occurs when the mechanical stress within the cap exceeds the mechanical strength of the plaque. The first studies on the stress distribution within the cap were performed by Richardson et al. (1989), Loree et al. (1992) and Cheng et al. (1993). There have been many subsequent studies which are reviewed in Cardoso and Weinbaum (2014). Figure 15.10 illustrates typical findings from computational stress modelling. The presence of the lipid pool leads to stress concentrations within the cap. The thickness of the fibrous cap also plays a role; thin caps have increased stress. This has led to the idea that vulnerable plaque (those with high risk of rupture) have high cap stress as a result of thin cap, whilst stable plaque (those with low risk of rupture) have low cap stress as a result of thick fibrous cap. Microcalcifications within the cap are also thought to play a role in that these act to locally increase the stress due to the differing gradients of stiffness between these microcalcifications and the surrounding fibrous tissue. Early attempts to quantify risk suggested that plaque with a cap thickness less than 65 μm were at risk of rupture (Loree et al. 1992), and that plaque with a peak wall stress greater than 300 kPa were at risk of rupture (Cheng et al. 1993).



Fig. 15.9
Constituents of an atherosclerotic plaque from a mechanical perspective

Fig. 15.10
Display of tissue stress (labelled circumferential stress) in a two-dimensional (2D) cross-section of a plaque. The 2D geometry was taken from microscopy of a section of an excised artery. Elastic moduli were taken from the literature and solid modelling used to estimate stress. Stress hot spots are shown at the shoulders of the fibrous cap. From Rohde L, Lee RT. Mechanical stress and strain and the vulnerable atherosclerotic plaque. In: Fuster V, editor. The vulnerable atherosclerotic plaque: understanding. Identification and modification. New York: Futura Publishing Company, 1999: p. 305–316. © Futura Publishing Company. Reprinted with permission from John Wiley & Sons
The material properties of the individual components of the plaque are required for stress modelling. Table 15.1 shows a summary of indicative values for elastic modulus of plaques as a whole and of the individual components in order to show differences in stiffness of the different components. In practice there are a wide range of values in the literature associated with the variability of plaques, the measurement conditions, and whether a linear or nonlinear stress-strain model is used. The overall stiffness of a plaque will strongly depend on the relative size of the lipid pool. Stable plaque with small or no lipid pool will be stiffer than vulnerable plaque with large lipid pool. This goes some way to explaining the large variation in the overall stiffness of plaque in Table 15.1.
Table 15.1
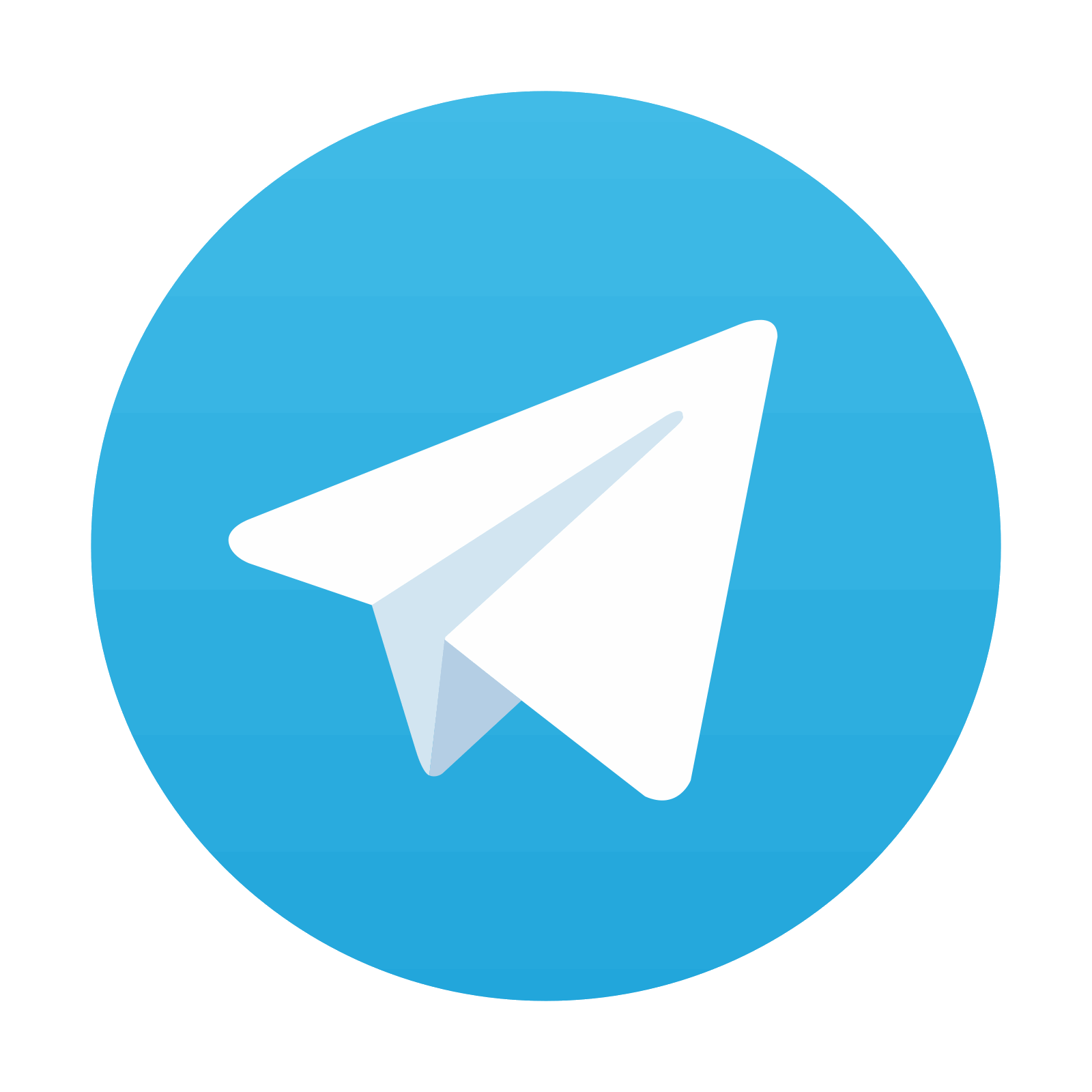
Elastic moduli of atherosclerotic plaque and plaque components; value (range)
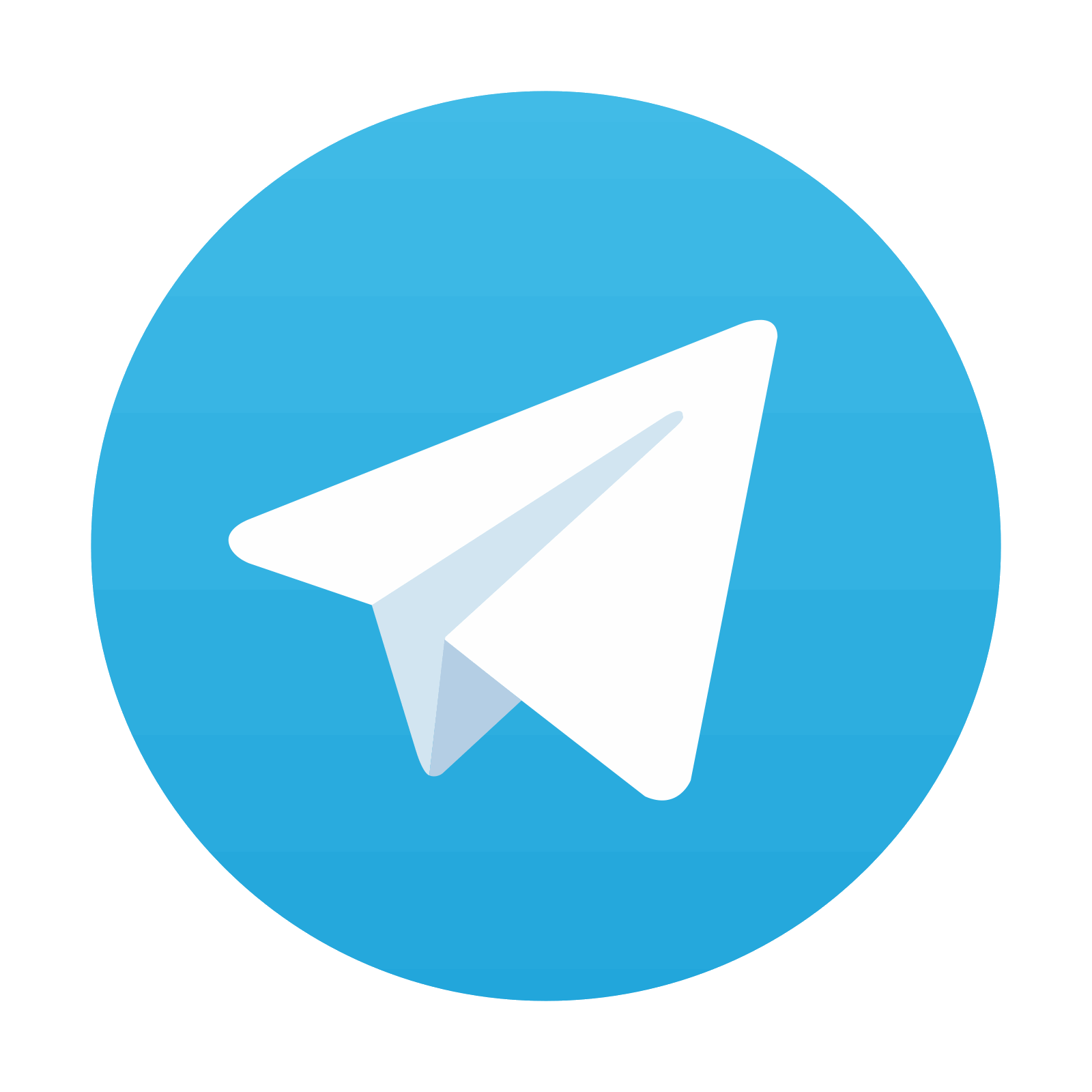
Stay updated, free articles. Join our Telegram channel
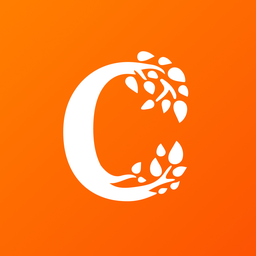
Full access? Get Clinical Tree
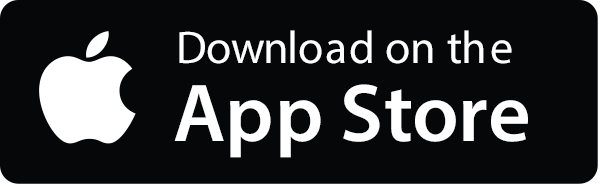
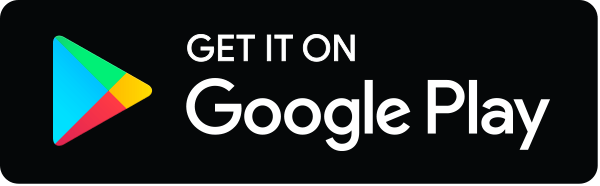
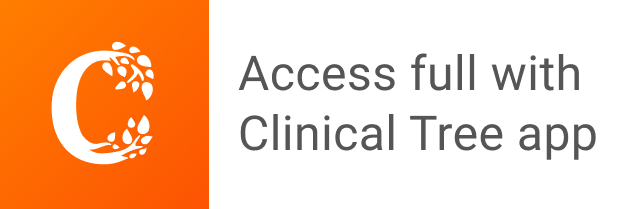