is important to distinguish between stunned and infarcted myocardium. First, the patient prognosis is changed. Several studies have shown that patients with acute ventricular dysfunction primarily resulting from myocardial necrosis have a worse prognosis than patients with ventricular dysfunction that is primarily reversible (11,12). Second, patient management during the acute setting may be changed. Viable but injured myocardium, such as stunned myocardium, is potentially at risk for future infarction if the reperfusion therapy was not complete and significant stenosis remains (11,13). In addition, a determination of the extent of nonviable and viable myocardium across the ventricular wall in a dysfunctional region may be valuable in selecting patients most likely to benefit from therapy that can modulate ventricular remodeling after acute myocardial infarction (MI), such as angiotensin-converting enzyme inhibitors and beta-blockers (14). Third, infarct size determined accurately in the acute setting may prove to be an adequate surrogate endpoint for the assessment of new therapies (15,16). For example, the efficacy of experimental therapies for acute coronary syndromes or acute MI could be evaluated without the need for “mega” trials with large sample sizes that use mortality as an endpoint. It is expected that the number of drug and device trials that employ cardiac magnetic resonance imaging parameters as a primary endpoint will increase substantially in the future (17).
![]() Figure 17.1. Clinical and physiologic markers to determine the size of infarction. (Adapted from Kaul S. Assessing the myocardium after attempted reperfusion: Should we bother? Circulation. 1998;98(7):625-627, with permission.) |
TABLE 17.1 Common Clinical Definitions of Myocardial Viability | ||||||||||||
---|---|---|---|---|---|---|---|---|---|---|---|---|
|
recovery sequence. From 1986 to 1999, image intensities in “hyperenhanced” regions were generally 50% to 100% higher than normal regions. The use of a segmented inversion recovery pulse sequence with the inversion time (TI) set to null signal from normal myocardium increased this differential approximately 10-fold to 1,080% in animals and 485% in humans (labeled “New Technique” in Table 17.2).
TABLE 17.2 Percentage Elevations in MR Signal Intensity of Infarcted Versus Normal Myocardium and Voxel Sizes: Previous Studies Compared With New Technique | ||||||||||||||||||||||||||||||||||||||||||||||||||||||||||||||||||||||||||||||||||||||||||||||||||||||||||||||||||||||||||||||||||||||||||||||||
---|---|---|---|---|---|---|---|---|---|---|---|---|---|---|---|---|---|---|---|---|---|---|---|---|---|---|---|---|---|---|---|---|---|---|---|---|---|---|---|---|---|---|---|---|---|---|---|---|---|---|---|---|---|---|---|---|---|---|---|---|---|---|---|---|---|---|---|---|---|---|---|---|---|---|---|---|---|---|---|---|---|---|---|---|---|---|---|---|---|---|---|---|---|---|---|---|---|---|---|---|---|---|---|---|---|---|---|---|---|---|---|---|---|---|---|---|---|---|---|---|---|---|---|---|---|---|---|---|---|---|---|---|---|---|---|---|---|---|---|---|---|---|---|---|
|
spatial resolution delayed enhancement images of the heart are obtained at the same slice locations as the cine images using a segmented inversion recovery fast gradient-echo (seg IR-FGE) pulse sequence. Figure 17.3 demonstrates cine and DE-MRI images from a typical patient scan. Each delayed enhancement image is acquired during an 8 to 10 seconds breath-hold, and the imaging time for the entire examination is generally 25 to 35 minutes.
![]() Figure 17.3. Images from a typical patient scan. Cine and delayed contrast-enhanced images are acquired at 6 to 8 short-axis locations and 2 to 3 long-axis locations during repeated breath-holds. Images are interpreted with cine images immediately adjacent to contrast images. This particular patient had a myocardial infarction caused by occlusion of the right coronary artery. Note hyperenhancement of the inferior wall. (From Kim RJ, Shah DJ, Judd RM. How we perform delayed enhancement imaging. J Cardiovasc Magn Reson. 2003;5(3):505-514, with permission.) |
![]() Figure 17.4. Timing diagram of two-dimensional segmented inversion recovery fast gradient-echo pulse sequence. ECG, electrocardiogram; α, shallow flip angle excitation; RF, radiofrequency; TD, trigger delay; TI, inversion time delay. Note that in this implementation, 23 lines of k-space are acquired every other heartbeat. See text for further details. (From Kim RJ, Shah DJ, Judd RM. How we perform delayed enhancement imaging. J Cardiovasc Magn Reson. 2003;5(3):505-514, with permission.) |
gadolinium dose, reaching 99% and 94% in acute and chronic MI, respectively, with a 0.3 mmol/kg dose (Fig. 17.5). Furthermore, with a dose of 0.2 mmol/kg, if MI was identified, it was in the correct location in more than 97% of patients. Clinically, we found that using doses as low as 0.10 to 0.15 mmol/kg still provides excellent image contrast between injured and normal myocardium, and reduces the time required to wait between intravenous contrast administration and delayed enhancement imaging (49,50,51 and 52). Sufficient time is required in order to allow the blood pool signal in the LV cavity to decline and provide discernment between LV cavity and hyperenhanced myocardium.
TABLE 17.3 Typical Parameters | ||||||||||||||||||||||||||||||||||||
---|---|---|---|---|---|---|---|---|---|---|---|---|---|---|---|---|---|---|---|---|---|---|---|---|---|---|---|---|---|---|---|---|---|---|---|---|
|
FOV phase than expected without resulting in wrap-around artifact over the heart; and (3) increasing the number of k-space lines acquired per cardiac cycle (i.e., segments) with the trade-off of worse temporal resolution, or with the same temporal resolution using a steady-state free precession (SSFP) instead of FGE readout (resultant shorter echo time [TE] and TR) at the expense of a reduction in pure T1 contrast effects. In some individuals, even with the approaches outlined here, there is still inadequate breath-holding. In this situation, the use of respiratory navigators may be helpful although imaging time is prolonged. Recently a fast, “single-shot” version of DE-MRI has been developed that can acquire snap-shot images during free breathing (53). This technique uses an SSFP readout with parallel imaging acceleration and provides complete LV coverage in less than 30 seconds. This technique could be considered the preferred approach in patients more acutely ill, unable to breath-hold, or with irregular heart rhythm. However, compared with standard, segmented DE-MRI, sensitivity for detecting MI is mildly reduced, and the transmural extent of infarction (TEI) may be underestimated (53,54).
previously stated, the total extent of hyperenhancement does not change. Lastly, if the TI is set far too short (Fig. 17.7A), infarct will be “nulled” and normal myocardium will hyper-enhance. Thus, it is far better to err on the side of setting the TI too long rather than too short.
![]() Figure 17.6. A: Inversion recovery curves of normal and infarcted myocardium assuming T1 of normal myocardium is 450 milliseconds and infarcted myocardium is 250 milliseconds. The time at which the magnetization of normal myocardium reaches the zero crossing is defined as the inversion time to “null” normal myocardium (312 milliseconds in this example). B: Image intensities resulting from an inversion prepulse with various inversion delay times. Note that image intensities correspond to the magnitude of the magnetization vector and cannot be negative. C: Difference in image intensities between infarcted and normal myocardium as a function of inversion time. The optimal inversion time is when the maximum intensity difference occurs. (From Kim RJ, Shah DJ, Judd RM. How we perform delayed enhancement imaging. J Cardiovasc Magn Reson. 2003;5(3):505-514, with permission.) |
![]() Figure 17.7. Delayed enhancement images in a subject with an anterior wall myocardial infarction in which the TI has been varied from too short to too long. See text for details. (From Kim RJ, Shah DJ, Judd RM. How we perform delayed enhancement imaging. J Cardiovasc Magn Reson. 2003;5(3):505-514, with permission.) |
with time, and the TI will need to be adjusted upward if delayed enhancement imaging is performed over a long time span (>5 minutes). For example, Figure 17.8
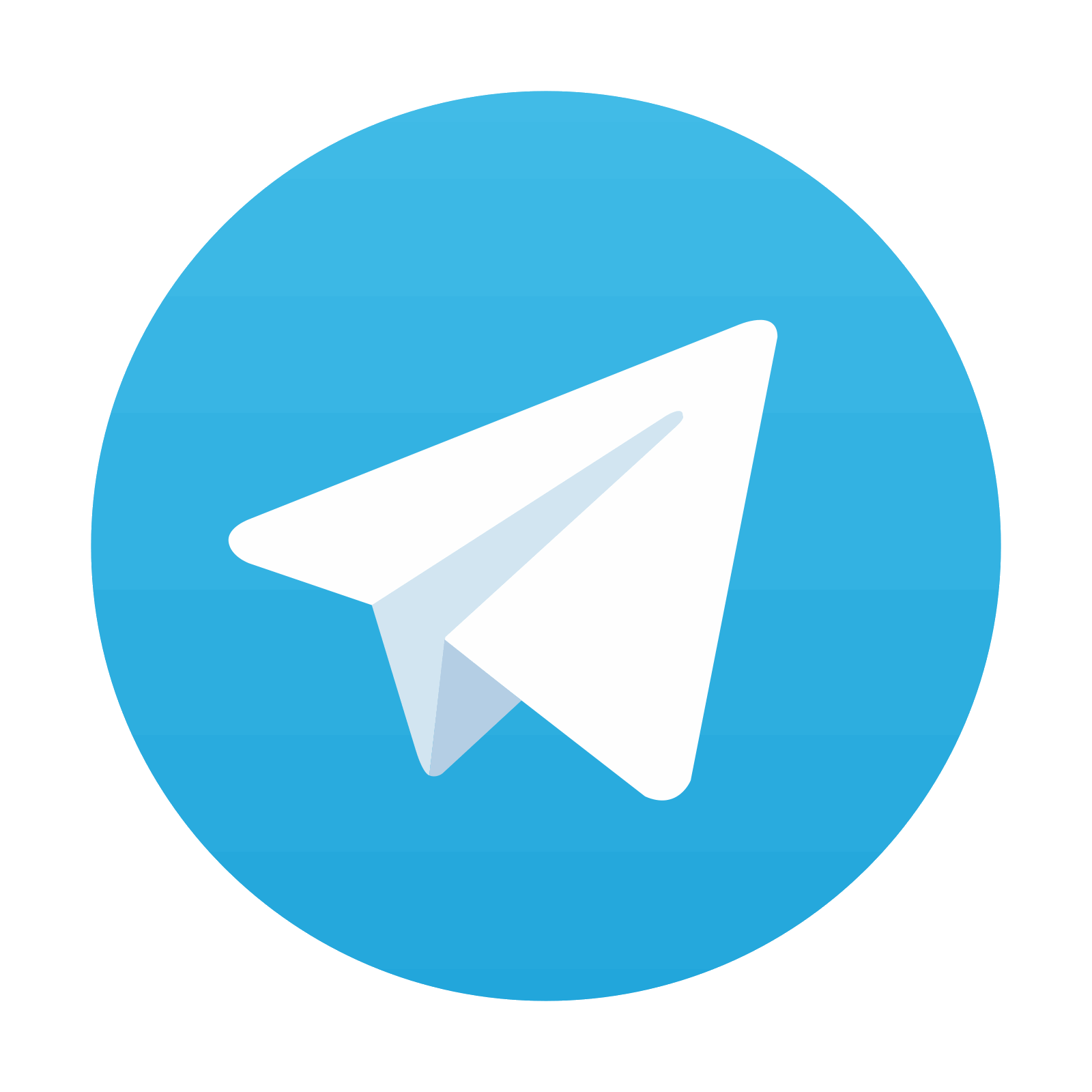
Stay updated, free articles. Join our Telegram channel
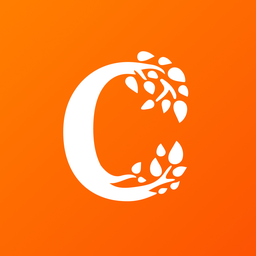
Full access? Get Clinical Tree
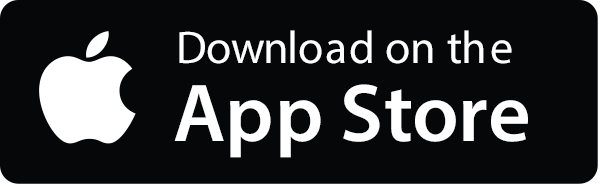
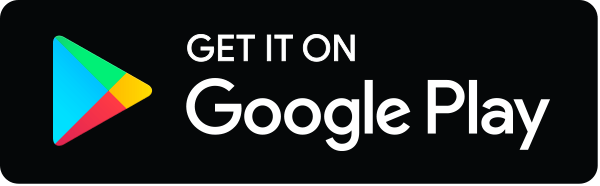
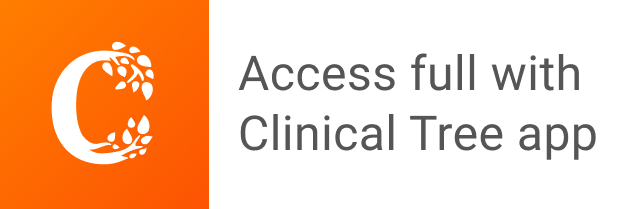