The complex molecular and cellular biology of the inflammatory process that promotes atherosclerosis has been elucidated through years of extensive experimental and clinical research. Vascular injury from inflammation initiates plaque formation and dictates the downstream clinical sequelae of atherosclerotic disease. It is essential that interventional cardiologists understand the natural history and pathologic processes of atherosclerosis, as well as the vascular biologic consequences of therapies employed during coronary intervention. This chapter describes (1) the pathophysiology of atherosclerosis; (2) the mechanisms responsible for an unstable plaque in acute coronary syndrome (ACS); and (3) the biology of vascular remodeling leading to restenosis, differences between balloon and stent injury, and therapies for restenosis.
Atherosclerosis is a result of risk factors and chronic arterial inflammation that promote sustained vascular injury.1 Several risk factors (Table 9-1) for atherosclerosis have been identified, including metabolic conditions such as sustained exposure to low-density lipoprotein (LDL) or hyperglycemia and insulin resistance associated with diabetes mellitus or metabolic syndrome. However, additional factors, including age, family history, physical factors (eg, uncontrolled hypertension resulting in changes in shear stress), environmental factors (eg, tobacco smoke), and infectious disease, also contribute to the development of atherosclerotic plaque. Vascular injury results from an inflammatory response that involves a complex sequence of interactions between endothelial and smooth muscle cells, leukocytes, inflammatory cells (eg, macrophages) and their secreted growth factors, and cytokines, which combine with lipoproteins and components of the vascular wall to form a mature atherosclerotic plaque. Inflammation plays a central role in pathogenesis of atherosclerosis; numerous studies have demonstrated a correlation between circulating inflammatory biomarkers (eg, C-reactive protein [CRP]) and an increased risk for atherosclerosis and adverse coronary events.2,3,4
Metabolic
|
Physical
|
Environmental
|
Infectious
|
Other
|
The biologic mechanisms in atherosclerotic plaque formation include intimal lipid accumulation, leukocyte recruitment, foam cell formation, neointimal growth, and vessel remodeling (Fig. 9-1).
FIGURE 9-1
Stages of atherosclerotic plaque growth. A. Initial stage of atherosclerosis involves injury to the vessel wall with subsequent expression of inflammatory adhesion molecules, which leads to leukocyte recruitment. B. Intermediate lesions involve macrophages imbibing oxidized low-density lipoprotein, leading to foam cell formation. There is continued leukocyte recruitment, formation of an early lipid core, and smooth muscle cell proliferation and migration. C. The advanced or mature atherosclerotic plaque consists of a necrotic lipid core with foam cells, necrotic debris, and free cholesterol esters. In addition, there is a fibrous cap consisting of smooth muscle cells and extracellular matrix. (From Ross R. Atherosclerosis—an inflammatory disease. N Engl J Med. 340:115-126. Copyright © 1999. Massachusetts Medical Society. Reprinted with permission from Massachusetts Medical Society.)

A key event in the early formation of atherosclerotic lesions is the accumulation of LDL within the arterial intima, which subsequently undergoes oxidation and glycation to initiate a cascade of molecular and cellular events including growth factor and cytokine release from inflammatory, endothelial, and smooth muscle cells. It is important to recognize that dyslipidemia results in an inflammatory state and is evident in the presence of inflammatory cells within the initial lesion of atherosclerosis, the fatty streak. Foam cells are the hallmark cell of the atherosclerotic lesion and consist of macrophages that are recruited to the subintima of the vessel wall and subsequently bind and internalize oxidized lipoprotein particles via a number of scavenger receptors on the cell surface. Importantly, lipoproteins can be cytotoxic to macrophages, leading to foam cell necrosis, which, in turn, causes necrotic debris and free cholesterol esters to accumulate within the lesion to form a necrotic core. Inflammatory cells, cytokines, and proteases weaken the fibrous cap surrounding the necrotic core, which can lead to atherothrombosis in the setting of a loss of integrity of the fibrous cap barrier, allowing contact of circulating blood with the thrombotic necrotic core.
Leukocytes, predominantly macrophages, play a pivotal role in atherosclerosis. By releasing cytokines and growth factors, macrophages regulate atherogenesis, but also influence plaque destabilization leading to rupture and thrombosis. The process of leukocyte recruitment, attachment to the extracellular matrix, and migration into the plaque (ie, diapedesis) is a response to vascular injury. Chemokine-stimulated endothelial cells express leukocyte-specific cell adhesion molecules (CAMs), which loosely bind circulating mononuclear cells and allow rolling of the monocytes along the endothelial surface.5 Tight binding of monocytes to the endothelium is mediated by the integrin class of CAMs, which is the final step prior to diapedesis. Although their pathologic role is uncertain, plasma levels of CAMs (eg, E-selectin or intercellular adhesion molecule-1 [ICAM-1]) are associated with the development of coronary atherosclerosis.6
Chemokines are an important group of cytokines produced by a number of cells (eg, smooth muscle cells, endothelial cells, and leukocytes) that act as a chemoattractant for leukocytes to areas of vascular injury. Two important chemokines are monocyte chemoattractant protein-1 (MCP-1) and interleukin (IL)-8, which participate in the recruitment of monocytes and diapedesis of adherent cells.7,8,9,10
Innate immunity and adaptive immunity have important roles in the development of atherosclerosis.11 Innate immunity is an immediate response to foreign material and relies on phagocytosis through neutrophils and monocytes. Monocytes are felt to be the first leukocytes recruited to the early atheromatous plaque and are differentiated into activated macrophages: type 1 induced by inflammatory cytokines (eg, interferon [IFN]-γ) or type 2 induced by IL-4, IL-13, and other anti-inflammatory cytokines. Type 1 macrophages produce large amounts of reactive oxygen species and inflammatory cytokines, which amplify the immune response to an atheroma. Type 2 macrophages express scavenger receptors and produce extracellular matrix proteins and remodeling stimuli.12,13,14 Other cells of the innate immune response have also been implicated in atherosclerosis, including neutrophils, mast cells, and natural killer cells.15 Adaptive immunity is an antigen-dependent response, and the CD4+ T lymphocyte is the primary cell present at atherosclerotic lesions. T cells actively secrete cytokines, which influence plaque progression and vulnerability.15 T lymphocytes secrete inflammatory cytokines such as IFN-γ, which can impair the extracellular matrix architecture and growth of smooth muscle cells, promote apoptosis, and cause plaque instability.16 CD4+ T cells also express the CD40 ligand to promote proteolysis through matrix metalloproteinases (MMPs) and stimulate cells to produce tissue factor to influence plaque rupture and thrombogenicity.17
As lesions mature, leukocytes accumulate in the “shoulder” regions of plaques (ie, the border between the eccentric plaque and normal vessel architecture), which are more vulnerable to plaque rupture.18 In addition, atherosclerotic lesions develop preferentially at coronary bifurcations. Due to the disturbances in flow patterns at coronary bifurcations, altered shear stress may upregulate CAMs and increase leukocyte recruitment and plaque growth. Monocytes contribute to vascular calcification in response to cytokines such as monocyte colony-stimulating factor (M-CSF) and receptor activator of nuclear factor κ-B (RANKL).19 Importantly, microcalcification is associated with plaque vulnerability.20
Smooth muscle cells and their secreted products are responsible for neointimal growth and for giving structure to the mature atherosclerotic plaque, which initially is a collection of lipids and foam cells. Growth factors and chemokines (eg, platelet-derived growth factor and thrombin) initiate smooth muscle cell migration from the media into the neointima and stimulate cell proliferation. In the neointima, smooth muscle cells expand the extracellular matrix by producing its constituent proteins including collagen, proteoglycans, elastin, fibrin(ogen), fibronectin, and vitronectin. The extracellular matrix accounts for a substantial portion of plaque volume and is vital to the structural integrity of the fibrous cap. Smooth muscle cells also express bone matrix proteins, which highlight their role in vascular calcification.21,22,23 Mineralization of the plaque occurs through deposition of calcium and osteopontin, but calcification does not always equate to plaque stability and is associated with higher risk in some patients (eg, elderly).24
Plaque angiogenesis is important to plaque growth and the pathogenesis of atherosclerotic complications.25 Angiogenic growth factors (eg, hypoxia-inducible factor [HIF] and vascular endothelial growth factor [VEGF]) stimulate the growth of vasa vasorum from the adventitia into the plaque.26 Notably, these vessels can hemorrhage, independent of plaque rupture, and extravasation of erythrocytes provides a source of cholesterol-rich red cell membrane constituents, heme, and iron, which act as stimulants for oxidative stress and vessel growth. Neovessel density is significantly higher in nonstenotic and stenotic noncalcified plaques compared with calcified lesions or vessels without plaque.27 Neoangiogenesis is driven by hypoxia, but the latter also contributes to proteolysis through MMPs. MMPs comprise a family of interstitial collagenases that weaken the fibrous cap and gellatinases that breakdown nonfibrillar collagen and the adhesions between endothelial cells, leading to plaque vulnerability.28,29,30 Hypoxia also promotes proinflammatory cytokine and leukotriene release, which activates macrophages and contributes to plaque growth.29,31
The mature atherosclerotic plaque is composed of a fibrous cap consisting of smooth muscle cells and extracellular matrix overlying a necrotic lipid core consisting of free cholesterol esters, foam cells, other leukocytes (eg, T cells), and necrotic debris from dead foam cells (see Fig. 9-1). Mature plaques are typically eccentric and heterogeneous, especially in terms of the thickness of the cap and distribution of leukocytes (highest at the shoulder regions of the plaque). These features are vitally important to plaque stability and the development of ACS.
A major limitation to coronary angiography is that it only provides information on the luminal encroachment of lesions. Intravascular imaging has provided a better understanding of atherosclerotic plaque architecture not only at sites of flow-obstructing lesions but throughout the vessel. Although the interventional cardiologist may be focused on focal obstructive lesions, it is important to realize that atherosclerosis is typically present throughout the entire coronary artery. The severity of lumen narrowing is subject to the amount of plaque growth and vascular remodeling, whereby the latter involves restructuring the cellular and noncellular components of the vessel wall under a variety of stressors (eg, smooth muscle mass increasing to normalize wall stress in hypertensive patients).32 Positive remodeling is a compensatory enlargement of the vessel to preserve the luminal area and maintain coronary blood flow (Fig. 9-2). MMPs are upregulated in areas of vessel wall remodeling and play a central role in plaque rupture.33
FIGURE 9-2
Schematic of vascular remodeling. As the atherosclerotic lesion progresses, initial enlargement of the entire vessel allows preservation of luminal area. As atherosclerosis becomes severe, enlargement is overcome by progression of the atherosclerotic plaque, and luminal area is compromised. (Adapted from Glagov S, et al. Compensatory enlargement of human atherosclerotic coronary arteries. N Eng J Med. 316: 1371-1375. Copyright © 1980. Massachusetts Medical Society. Reprinted with permission from Massachusetts Medical Society.)

The clinical manifestations of coronary artery disease are often described as a spectrum, from stable angina associated with exertional angina and benign outcomes, to ST-segment elevation myocardial infarction (STEMI) associated with sudden thrombotic vessel occlusion and higher rates of morbidity and mortality. Two main mechanisms are primarily responsible for the clinical manifestations of atherosclerosis: (1) luminal narrowing that leads to a mismatch between oxygen supply and demand typically resulting in symptoms of stable angina; or (2) atheromatous plaque rupture resulting in thrombus formation and coronary occlusion.34 Importantly, atherosclerosis can also alter the normal endothelial vasomotor function and autoregulation of blood flow (ie, endothelial dysfunction), which can cause anginal symptoms in patient with and without epicardial coronary stenoses. Nevertheless, it is critical to recognize that thrombotic complications of atherosclerosis depend on plaque morphology and vascular biologic factors, not the severity of coronary stenosis.
Lumen encroachment occurs from the growth and expansion of atherosclerotic lesions (see Fig. 9-2). The extent of luminal narrowing depends on the size of the atherosclerotic lesion and the amount of compensatory vascular remodeling. A coronary stenosis reduces coronary blood flow to a given vascular territory. The decrease in blood flow causes the distal microcirculation to vasodilate and increase coronary blood flow, which, in turn, reduces the ability of the coronary circulation to increase blood supply in response to demand (ie, coronary flow reserve). This process typically leads to exertional angina and is relieved by rest. Importantly, luminal encroachment does not always cause symptoms and depends on many factors including the severity of the stenosis, the oxygen carrying capacity of blood, and the supply demands of the distal myocardial bed.
A general rule is that lesions typically produce symptoms when they reach a 60% to 70% diameter stenosis. However, interrogating the intracoronary hemodynamics with flow and pressure wires has revealed that lesions with the same degree of angiographic stenosis may have very different hemodynamic and ischemic consequences.35 Fractional flow reserve (FFR) has become the predominant method used to assess coronary hemodynamics and is defined as the ratio of the distal pressure in the coronary artery beyond the lesion divided by the aortic pressure. Based on an association with inducible ischemia on stress testing, the initial cut-off point for not performing percutaneous coronary intervention (PCI) after FFR assessment was <0.75, as established in the FFR to Determine Appropriateness of Angioplasty in Moderate Coronary Stenoses (DEFER) study.36 To enhance the sensitivity and exclude ischemia using FFR, the cut-off was increased to >0.80 in the Fractional Flow Reserve Versus Angiography for Multivessel Evaluation (FAME) trial.37,38 In FAME, routine measurement of the FFR was compared with angiography alone for guiding PCI in patients with multivessel coronary artery disease (CAD). The use of FFR significantly reduced the rate of major adverse cardiac events compared with angiography alone.37,39 Importantly, a subgroup analysis of the lesions that were assessed by FFR in FAME revealed that coronary angiography could not accurately predict the hemodynamic significance of a coronary lesion.40 In FAME, 35% of lesions with an angiographic stenosis of 50% to 70% (ie, intermediate) were hemodynamically significant (FFR ≤0.80), whereas 20% of lesions with an angiographic stenosis of 71% to 90% were not functionally significant by FFR.40 Only when lesions had an angiographic stenosis >90% was coronary angiography able to predict hemodynamic significance, where 96% of lesions with a coronary stenosis of 91% to 99% had an FFR ≤0.80.40 Furthermore, 54% of patients who were classified as having multivessel CAD did not have ≥2 lesions with an FFR ≤0.80. The discordance between hemodynamic significance and angiographic stenosis highlights the need to use adjunctive techniques to assess the ischemic potential of coronary stenoses.
The historical view held that stable angina could convert to ACS when an atherosclerotic lesion narrowed the vessel lumen to a critical point where vasospasm or thrombosis in situ could develop and cause a myocardial infarction (MI). For years, considerable debate ensued as to whether thrombus found at autopsy was a pre- or postmortem phenomenon. This widely held view continued despite James Herrick’s landmark publication of thrombus as the predominant cause of sudden coronary obstruction in 1912.41 The pivotal work by DeWood et al42 in 1980 demonstrated angiographically that ST-segment elevation and transmural MI were associated with occlusion of an epicardial coronary vessel predominantly secondary to thrombus (Fig. 9-3). Since this discovery, autopsy studies and angioscopy have confirmed the presence of visible thrombus associated with plaque rupture (Fig. 9-4) in both unstable angina and acute MI (AMI).42
FIGURE 9-3
Percentage of vessels totally occluded in patients presenting after acute myocardial infarction as a function of time after onset of symptoms. (Adapted from DeWood MA, et al. Prevalence of total coronary occlusion during the early hours of transmural myocardial infarction. N Engl J Med. 303:897-902. Copyright © 1980. Massachusetts Medical Society. Reprinted with permission from Massachusetts Medical Society.)

FIGURE 9-4
Histologic example of a ruptured plaque with subsequent thrombosis leading to a fatal myocardial infarction. (From Constantinides P. Plaque hemorrhages, their genesis and their role in supra-plaque thrombosis and atherogenesis. In: Glagov S, Newman WP III, Schaffer SA, eds. Pathology of the Human Atherosclerotic Plaque. New York: Springer-Verlag; 1990:393-411, with permission of Springer.)

Early thrombolytic trials were instrumental in our current understanding of ACS. As part of these trials, patients with AMI underwent serial angiography after randomization to thrombolytics or placebo, which revealed an unexpected finding, namely that the majority of the culprit lesions had <50% in diameter stenosis (Fig. 9-5). Furthermore, in some patients, mild and moderate stenoses progressed to MI within weeks.43,44 Importantly, only 15% of AMIs arose from lesions with a coronary stenosis >60% on a prior angiogram (see Fig. 9-5).44 These finding focused future research efforts on determining the vascular biology of a vulnerable plaque and the mechanisms responsible for conversion from a stable plaque to a thrombotic lesion. Noncritical lesions are significantly more abundant than critical lesions, and compensatory enlargement of the vessel often accompanies atherosclerosis. Thus, mildly stenotic lesions can have an even larger plaque burden by volume, which may portend a higher risk for plaque rupture and thrombosis.
FIGURE 9-5
Compiled data from 4 thrombolytic trials showing that the majority of underlying lesions responsible for acute myocardial infarction involve less than 50% diameter stenosis. (Reproduced with permission from Smith SC. Risk-reduction therapy: the challenge to change. Circulation. 1996;93:2205-2211.)

Plaque rupture is the proximate event leading to thrombosis, which exposes the subendothelium to circulating blood. Several histologic features have been associated with plaque vulnerability, including a thin fibrous cap, a large lipid-laden necrotic core, and an accumulation of leukocytes and inflammatory cells at the shoulder regions of the plaque (Fig. 9-6).18 An important natural history study of coronary atherosclerosis was recently conducted called Providing Regional Observations to Study Predictors of Events in the Coronary Tree (PROSPECT).45 In PROSPECT, 697 patients who were stented in the setting of ACS underwent 3-vessel intravascular imaging to determine the clinical and lesion risk factors associated with future events.45 At 3 years, approximately 20% of patients experienced a major adverse cardiac event, in which nearly 50% of the adverse events were associated with nonculprit lesions.45 The majority of adverse cardiac events were in lesions that were mildly stenotic at the time of index angiography.45 Three lesions characteristics were strongly associated with a higher risk for future adverse cardiac events in nonculprit lesions; these were a plaque burden ≥70%, presence of a thin cap fibroatheroma, and a minimal lumen area ≤4 mm2.45
FIGURE 9-6
Characteristics of stable versus vulnerable plaques. Vulnerable plaques have thinner fibrous caps and larger, more inflammatory cell–rich lipid cores. SMC, smooth muscle cell. (Reproduced with permission from Libby P. Molecular bases of the acute coronary syndromes. Circulation. 1995;91:2844-2850.)

A plaque’s structural integrity is regulated by vascular inflammation and dependent on the balance between the mass of smooth muscle cells and content of the extracellular matrix. Smooth muscle cell mass is regulated by cell migration from the media, neointimal proliferation, and cell death. The latter occurs due to cytokine release from inflammatory cells, leading to apoptosis.46 Extracellular matrix content depends on the interplay between production from smooth muscle and inflammatory cells and protease activity leading to degradation (Fig. 9-7). Within a plaque, activated T cells release IFN-γ, which inhibits smooth muscle cell collagen synthesis. Inflammatory cells also produce enzymes (eg, MMPs and cathepsins) that degrade important structural components of the extracellular matrix (ie, collagens, elastin).18 This process weakens the structural integrity of a plaque by decreasing smooth muscle cell mass and degrading the extracellular matrix.
FIGURE 9-7
Thickness of the fibrous cap is a balance between synthesis of extracellular matrix proteins by smooth muscle cells (SMCs) and the breakdown of these products by degradative enzymes. These processes are largely under the influence of inflammatory cells. IFN, interferon; MCP-1, monocyte chemoattractant protein-1; M-CSF, monocyte colony-stimulating factor; TNF, tumor necrosis factor. (Reproduced with permission from Libby P. Molecular bases of the acute coronary syndromes. Circulation. 1995;91:2844-2850.)

Predicting how, why, and when a plaque will rupture is a subject of continuing study. An interesting historical observation is the diurnal variation in MI presentation, which peaks in the early morning hours.47 Additionally, MI rates increase during times of extreme stress (eg, earthquake).48 These observations suggest that variations in cortisol and adrenaline levels may impact plaque rupture through their systemic hemodynamic effects. The site of rupture coincides with the highest circumferential biomechanical force, which is located at the shoulder region of a plaque.49 Thus, a combination of biochemical and biophysical characteristics predispose the shoulder regions to plaque rupture, which is supported by histologic postmortem studies in patients who died from an AMI. Other processes may also be responsible for the thrombotic complications of ACS. Focal endothelial denudation can expose the internal elastic membrane to circulating blood and act as a substrate for thrombosis, which occurs more frequently in women and diabetics. Also, mechanical injury during PCI can also disrupt plaque and lead to acute thrombosis.
Atherothrombosis is the final consequence of plaque rupture or endothelial denudation that leads to acute coronary vessel occlusion. Exposing the lipid core (ie, tissue factor associated with lipid-laden and necrotic macrophages) to circulating blood is a potent stimulus for thrombus formation. Thrombus formation following plaque rupture is regulated by the blood’s tight control over the balance between procoagulant-anticoagulant and fibrinolytic-antifibrinolytic factors. In the presence of an intact and robust fibrinolytic system, a thrombus may undergo rapid lysis and manifest clinically as unstable angina or non–ST-segment elevation MI (NSTEMI). Similarly, patients on antiplatelet therapy (eg, aspirin) may be protected from platelet activation and aggregation during subendothelial exposure to blood. Conversely, the presence of prothrombotic factors (eg, fibrinogen or plasminogen activator inhibitor-1) can accelerate the growth of a thrombus, leading to coronary occlusion. Nonocclusive thrombus can also become incorporated into a plaque during the healing process and provide a mechanism for plaque expansion and lumen encroachment and be a source for angina.
Importantly, antiplatelet and lipid-lowering therapy trials have corroborated the thrombotic paradigm of ACS and demonstrated marked reductions in the morbidity and mortality associated with an acute coronary event. Furthermore, these breakthroughs have been achieved despite a lack of change in lesion severity.44 Lipids have a central role in atherosclerosis and atherothrombosis as the critical initiating and sustaining inflammatory stimulus for plaque growth and rupture. The beneficial actions of statins include not only their lipid-lowering properties, but also the reduction in inflammation, which can stabilize the fibrous cap and reduce thrombogenicity of the inner lipid-laden necrotic core. Evidence supports that lipid-lowering therapy is vital to acute and chronic therapy for patients presenting with ACS.
A major goal of vascular biologists and clinical cardiologists is to develop a more complete understanding of the mechanisms of plaque rupture and the development of novel strategies to stabilize lesions. However, many different lesions of varying vulnerability may coexist throughout the coronary tree. Novel imaging techniques are being developed to identify plaques that are the most susceptible to rupture, which may allow for better prognostication and therapeutic developments.
Andreas Gruentzig ushered in the modern era of management of obstructive CAD with the publication of his experience with coronary balloon angioplasty in a landmark study of 5 patients in 1978.50 Yet, his first angiographic follow-up study revealed that 19% of patients undergoing successful initial angioplasty suffered restenosis.51 Since the early days of balloon angioplasty, the introduction of stenting has reduced certain limitations of balloon angioplasty. However, in-stent restenosis (ISR) still remains an important limitation of coronary stents. Bare metal stents (BMS) decreased restenosis compared to balloon angioplasty, but in selected studies, ISR remained as high as 30%.52 Drug-eluting stents (DES) further reduced the rates of ISR to as low as 5% to 20%, depending on the patient population.53,54,55,56
Our understanding of the pathophysiology of restenosis has evolved considerably. Early postmortem studies revealed a fibrocellular response at sites of prior balloon angioplasty.57 Initial animal studies revealed that endothelial denudation, medial dissection, and platelet deposition contribute to an immediate response to balloon injury, whereas late restenosis is a consequence of smooth muscle cell migration and proliferation.58,59 The initial paradigm for restenosis comprised 3 phases: (1) an inflammatory phase, (2) a granulation or cellular proliferation phase, and (3) a phase of remodeling involving extracellular matrix protein synthesis (Fig. 9-8).60 As with atherosclerosis, inflammation plays a critical role in the pathogenesis of restenosis. Coronary stenting has a profound impact on the vascular biologic response to vessel injury, especially the inflammatory response and vascular remodeling following injury. The aim of this chapter is to understand the pathogenesis of restenosis and the clinical indicators and biochemical markers associated with increased risk and to introduce the concepts of antirestenotic therapy.
FIGURE 9-8
A. A mature atherosclerotic plaque prior to intervention. B. The immediate result of stent placement with endothelial denudation and platelet/fibrinogen deposition. C and D. Leukocyte recruitment, infiltration, and smooth muscle cell (SMC) proliferation and migration in the days following injury. E. Neointimal thickening in the weeks following injury with continued SMC proliferation and monocyte recruitment. F. The long-term (weeks to months) change from a predominantly cellular to a less cellular and more extracellular matrix (ECM)-rich plaque. FGF, fibroblast growth factor; IGF, insulin-like growth factor; IL, interleukin; MCP-1, monocyte chemoattractant protein-1; PDGF, platelet-derived growth factor; TGF-β, transforming growth factor-β; VEGF, vascular endothelial growth factor. (Reproduced with permission from Welt FGP, Rogers C. Inflammation and restenosis in the stent era. Arterioscler Thromb Vasc Biol. 2002;22:1769-1776.)

In clinical trials, restenosis was historically defined angiographically as a binary (ie, yes or no) reduction of ≥50% of the luminal diameter compared with the reference vessel, which does not always separate clinically relevant lesions from those without any sequelae.61 However, restenosis can also be defined by angiographic terms that are more relevant to our understanding of the pathophysiology. Late lumen loss (LLL) is the continuous measure of angiographic luminal narrowing and is calculated by subtracting minimal lumen diameter (MLD) at the time of index revascularization from postprocedural MLD at angiographic follow-up. LLL is an important surrogate marker used to assess the effectiveness of antirestenotic therapies and different stent platforms.62,63 It is important to note that virtually all patients have some degree of restenosis. In any given population, the severity of restenosis will follow a typical Gaussian distribution (Fig. 9-9), and only a minority of patients will have severe ISR (eg, diameter stenosis >70%) and anginal symptoms. On average, LLL is 0.8 to 1.0 mm following implantation of a BMS, regardless of the reference vessel diameter, and is critically important when considering revascularization of smaller vessels. For example, a 4-mm diameter vessel would lose 44% of lumen area with an LLL of 1 mm, whereas a 2-mm vessel with the same 1-mm loss would have a 75% loss in lumen area. LLL and angiographic restenosis are related to the reference vessel diameter, and restenosis is heavily dependent on the definition used in a given clinical study.64 An inventory of the wide variety of angiographic and clinical definitions used to define restenosis is shown in Table 9-2.
Binary markers
|
Continuous markers
|
FIGURE 9-9
Histogram of percent diameter stenosis at follow-up angiography of 1445 lesions treated with percutaneous intervention. The superimposed curve represents the theoretic Gaussian distribution. SD, standard deviation. (Reproduced with permission from Rensing BJ, Hermans WR, Deckers JW, et al. Lumen narrowing after percutaneous transluminal coronary balloon angioplasty follows a near gaussian distribution: a quantitative angiographic study in 1,445 successfully dilated lesions. J Am Coll Cardiol. 1992;19:939-945.)

The discordance between LLL and binary restenosis is controversial. Late loss is a useful surrogate marker for assessing the effectiveness of therapies for restenosis. However, the relationship between late loss and binary restenosis is nonlinear and a curvilinear function (Fig. 9-10), which implies that there may be a threshold of LLL that is clinically significant. The argument is that LLL below a certain level is not clinically significant and does not portend a worse clinical restenosis rate (ie, target lesion revascularization [TLR]) (see Fig. 9-10). This argument is a contentious issue in interventional cardiology. Regardless, the clinical sequelae of angiographic restenosis are not always concordant. An example of this was observed in a meta-analysis of patients with angiographic ISR from multiple stenting trials, where ISR defined as ≥50% diameter stenosis was asymptomatic in approximately 50% of patients.65 This study highlights the multifactorial nature of restenosis and discrepancy between angiographic restenosis as a binary outcome and corresponding clinical significance.
FIGURE 9-10
A. Data from the TAXUS IV trial shows the in-stent late loss for the Taxus stent (Boston Scientific) and control bare metal stent. B. Probability of target lesion revascularization (TLR) as a function of in-stent late loss revealing a curvilinear distribution. Superimposed are the mean and standard deviations for the Taxus stent late loss (red) and the control bare metal stent (blue). The distribution of the Taxus stent late loss falls along the relatively flat portion of the curve, whereas the distribution for the bare metal stent falls along the steeper portion of the curve where there is greater correlation between late loss and TLR. (Reproduced with permission from Ellis SG, Popma JJ, Lasala JM, et al. Relationship between angiographic late loss and target lesion revascularization after coronary stent implantation: analysis from the TAXUS-IV trial. J Am Coll Cardiol 2005;45:1193-1200.)

Traditionally, restenosis has been viewed as a condition that clinically manifests as stable angina. However, there is substantial evidence to the contrary. Although the majority of patients present with stable exertional symptoms, unstable angina (26%-53%), and acute MI (3.5%-20%) are not uncommon.66,67 Restenosis following balloon angioplasty or stenting is an accelerated process compared with atherosclerosis. With balloon angioplasty, clinically significant restenosis is typically present by 6 months.68,69 Following stent implantation, the average time for ISR is approximately 5.5 months, with evidence that a shorter interval may be present in patients presenting with AMI.70 Taken together, these studies highlight that restenosis is not always a benign condition and is a distinct clinical entity from atherosclerosis.
The 3 most important and historical clinical risk factors for restenosis are stent length, MLD, and diabetes mellitus.71 In conceptualizing the risk factors for restenosis, it is helpful to classify them as biologic or mechanical, although there is considerable overlap between the 2 classifications.
The risk for restenosis is high among patients with diabetes mellitus. Data from a registry involving >35,000 patients following DES (Endeavor, sirolimus-eluting stents [SES], Taxus Express, and Liberte) revealed that, at 2-year follow-up, the rates of ISR with DES implantation were significantly higher in patients with diabetes.72 Another study of 954 patients undergoing PCI revealed that TLR was also significantly higher in diabetics compared with individuals without diabetes.73 The increased risk for restenosis in diabetics is likely secondary to endothelial dysfunction, accelerated intimal hyperplasia, and increased platelet reactivity.74
Patients may also be genetically predisposed to restenosis. For example, genetic polymorphisms associated with a higher risk for restenosis include genes for angiotensin-converting enzyme inhibitor, glycoprotein receptor IIIa PLA1/2, haptoglobin 2/2.25, and IL-8.75,76 Additionally, resistance to antiproliferative drugs used in DESs has been observed in patients with genetic polymorphisms to the intracellular receptor mammalian target of rapamycin (mTOR), enzymes responsible for paclitaxel or sirolimus metabolism, and mutations of proteins in the downstream signaling of mTOR.77,78 Thus, biologic resistance to DESs may be presents in certain individuals.
Biomarkers associated with an increased risk for ISR have also been identified. Higher plasma levels of MCP-1 have been associated with a higher rate of restenosis at 6-month angiographic follow-up following balloon angioplasty.79 CRP is considered a strong risk predictor for cardiovascular disease, but the relationship between CRP and ISR remains controversial and an area of active investigation.80,81,82,83,84 Circulating MMPs have also been associated with a higher risk of developing ISR following DES implantation. MMP-2 and MMP-9 play a key role in the migration of vascular smooth muscle cells and remodeling following vascular injury. Significant elevations in MMP-2 and MMP-9 levels are strongly associated with the development of ISR following DES implantation.85
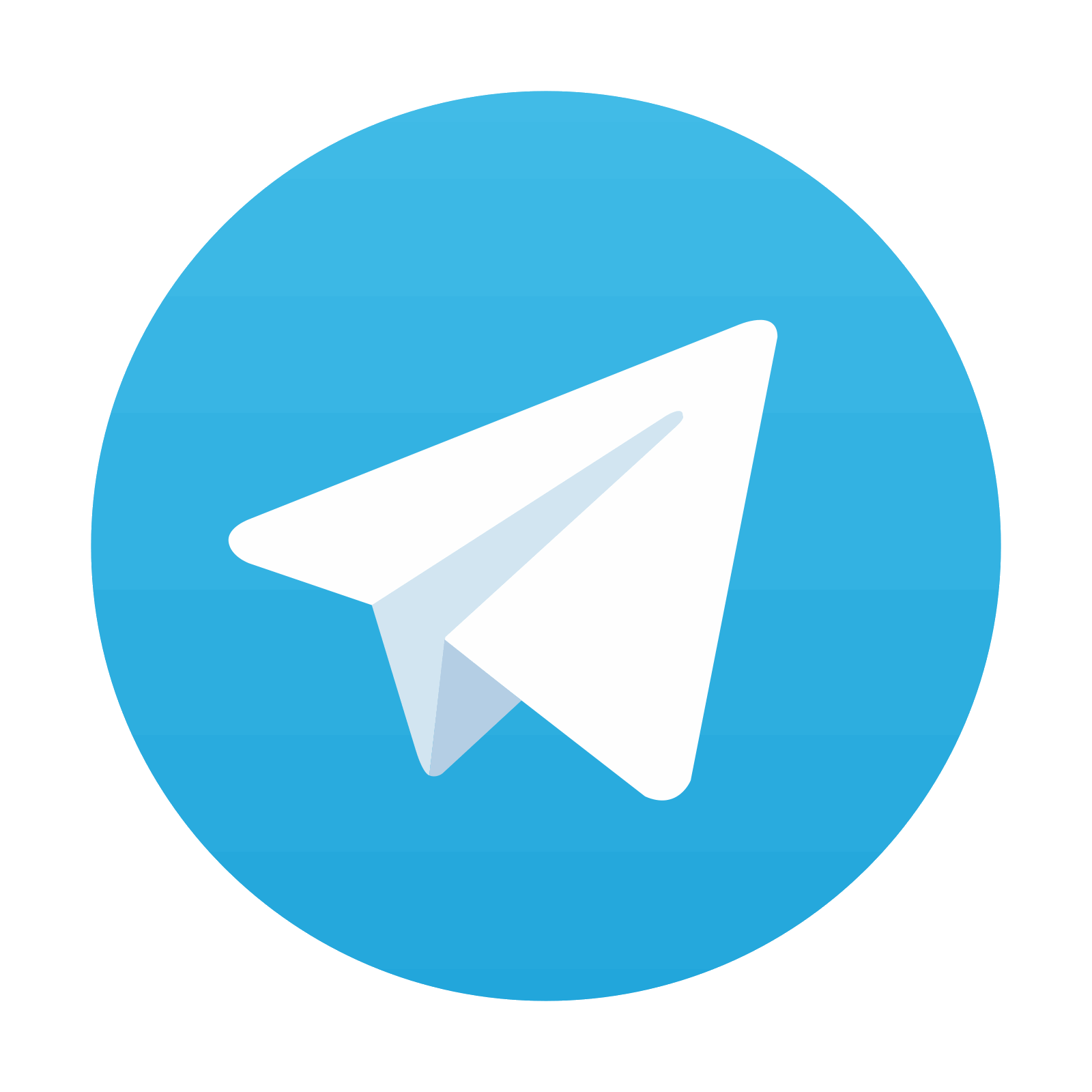
Stay updated, free articles. Join our Telegram channel
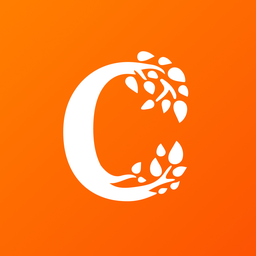
Full access? Get Clinical Tree
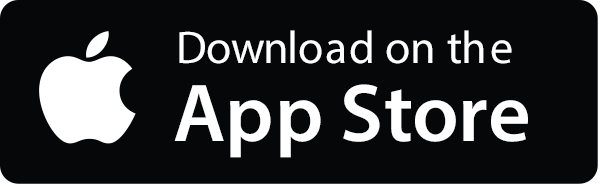
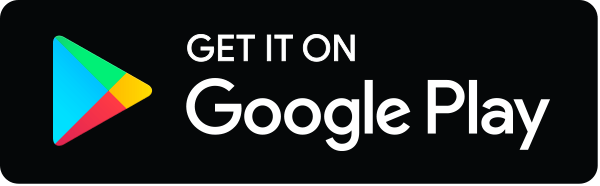