Background
Speckle-tracking enables direct tracking of carotid arterial wall motion. Timing intervals determined with carotid speckle-tracking and slopes calculated from carotid artery area versus cardiac cycle curves may provide further information on arterial function and stiffness. The proposed arterial stiffness parameters were examined in healthy controls ( n = 20), nondiabetic patients with hypertension ( n = 20), and patients with type 2 diabetes ( n = 21).
Methods
Bilateral electrocardiographically gated ultrasonograms of the distal common carotid artery were acquired using a 12-MHz vascular probe. Four timing intervals were derived from speckle-tracked carotid arterial strain curves: (1) carotid predistension period, (2) peak carotid arterial strain time, (3) arterial distension period, and (4) arterial diastolic time. In addition, carotid artery area curves were recorded over the cardiac cycle and subdivided into four segments, S1 to S4, relating to arterial distention and contraction periods.
Results
Mean far wall predistension period and peak carotid arterial strain time were more delayed in patients with diabetes and hypertension than in controls. Global mean arterial distension period was prolonged and arterial diastolic time was shorter in patients with hypertension and diabetes than in controls. Slopes of segments S2 and S4 were markedly steeper in the combined group of patients with hypertension and diabetes compared with healthy controls ( P = .03 and P = .02, respectively).
Conclusions
Speckle-tracking-based measures of arterial stiffness may provide potential additive value in assessing vascular function in patients at risk for cardiovascular disease.
Carotid arterial strain (CAS) measured using ultrasound-based distensibility is a method for assessing local arterial stiffness. Distensibility-based CAS measures have been shown to be associated with risk factors for cardiovascular disease and with incident stroke. More recently, along with other groups, we reported that ultrasound-based CAS can be measured using speckle-tracking, that CAS measured using speckle-tracking was lower in subjects with diabetes mellitus (DM), and that this may be a more sensitive measure than conventional local measures of CAS. In addition to measuring CAS, speckle-tracked strain curves can be used to provide other information, such as the timing of various events (e.g., time from ventricular systole to the onset of arterial expansion, time to peak arterial expansion, time spent in expansion and recoil, and the rate of these changes) in the arterial wall over the course of the cardiac cycle. Noninvasive measures of cardiac time intervals have been shown to have value in assessing cardiac function. However, limited data exist with respect to the importance of timing intervals in the evaluation of arterial health.
We aimed to determine if (1) CAS measured by speckle-tracking, which we have shown to be lower in patients with diabetes, is also lower in subjects with hypertension compared with healthy individuals and (2) the durations of various events in the arterial cycle (expansion and recoil) and (3) the slopes of carotid artery (CA) area (CAA) over the cardiac cycle, as obtained with speckle-tracking, can provide additional information on vascular function as assessed by CAS. Additionally, we evaluated if the above parameters were related to hypertension and DM, disease states known to be associated with increased arterial stiffness.
Methods
Study Population
Subjects for our present analysis were selected from an ongoing study whose aim is to describe CAS in various states of health. From a total of 102 individuals who were recruited at the noninvasive cardiac laboratory of our local county hospital (Ben Taub General Hospital, Houston, TX), we selected 21 individuals with DM, 20 patients with hypertension, and 20 healthy controls such that they were matched for age and gender. The differences in CAS between individuals with DM and healthy controls have previously been published. Hypertension was defined as presence of International Classification of Diseases, Ninth Revision, code 401.0 in the subject’s medical problem list or the active use of antihypertensive medications. Subject recruitment and carotid ultrasound were performed at any time during the day, with no consideration given to intake of food, caffeine, alcohol, tobacco, or vasoactive medications, because we believe this scenario represents conditions encountered more typically in a clinical setting. Exclusion criteria included known carotid stenosis >70%, history of carotid stenting or carotid endarterectomy, history of radiation therapy to the neck, clinically significant aortic valve disease, any persistent arrhythmia, or a known life expectancy <6 months. The study was approved by the local institutional review board, and written informed consent was obtained from all participants.
Ultrasound Image Acquisition
The acquisition of carotid ultrasound images and segmental carotid strain analyses were conducted as previously described. All subjects were interviewed and scanned by the same study investigator. Briefly, the distal common CA was imaged 1 cm inferior to the carotid bulb (mean frame rate, 77.6 ± 7.9 frames/sec) in transverse section with electrocardiographic gating over three cardiac cycles using a 14-MHz M12L vascular transducer (Vivid 7; GE Healthcare, Waukesha, WI). Images were exported in Digital Imaging and Communications in Medicine format for offline analysis, with each subject assigned a unique code.
CAS Analysis
Ultrasound scans were uploaded to a computer workstation equipped with a speckle-tracking software package (2D Cardiac Performance Analysis, TomTec Imaging Systems GmbH, Munich, Germany). TomTec provided the analysis software but otherwise had no input into the study design, data accrual, analysis, or manuscript preparation. Details of the CAS analysis by speckle-tracking have been previously published. Briefly, circumferential CAS measures were obtained in six segments as in the myocardial short-axis views in segments of the distal common CA free of plaque. Arterial segments located anteriorly ( n = 3) were identified as near wall segments and those inferiorly as far wall segments ( n = 3). Peak systolic circumferential strain values were measured and averaged (i.e., mean of the peak) for all six segments and then separately for the three far wall segments to yield a global net and far wall average CAS, respectively. We have reported that these two measures have the greatest intervisit, interreader, and intrareader reproducibility. These values were averaged over the three cardiac cycles and over both the left and right sides to obtain one net global and far wall CAS average per individual. Luminal CAS (i.e., the traditional method) was measured using maximum and minimum carotid luminal diameters averaged over 1 cm of the longitudinal distal common CA, 1 cm inferior to the carotid bulb.
Time Intervals
Four time intervals were measured. The first measure was the time from start of electrical systole (i.e., the start of the QRS complex on electrocardiography) to the time at the peak CAS value (i.e., peak CAS time; segment A in Figure 1 ). However, after the start of electrical cardiac systole, the CA continued to contract to a minimum value ( Figure 1 ; because of the delay between electrical cardiac systole and CA systole). This time interval, between the onset of electrical systole and the CAS minimum, was called the predistension period (PDP; segment B in Figure 1 ). The time difference between the measured peak CAS time and PDP constituted the arterial distension period (ADP; segment C in Figure 1 ). Finally, the arterial diastolic time was calculated as the time from peak CAS to the end of the cardiac cycle (segment D in Figure 1 ).

The PDP includes the electrical-mechanical delay in the onset of myocardial systole, the isovolumic contraction period, the mechanical systole of the heart, and, finally, the propagation of the pulse through the arterial tree to the distal common CA. Although the isovolumic contraction time can vary depending on the afterload experienced by the heart, in general, it increases with increasing myocardial stiffness (i.e., takes more time). On the other hand, stiffer vessels mean faster propagation of the pulse wave (i.e., less time). The PDP therefore included information related to both myocardial and the arterial tree’s performance but may be difficult to interpret because of the opposite effects on the isovolumic contraction time and pulse-wave propagation. The ADP, on the other hand, represents the time taken for the vessel to go from its minimum to its maximum dimension and would be a function of the local pressure driving arterial distension and the intrinsic stiffness of the artery. The peak CAS time is a combination of both PDP and ADP. Finally, the arterial diastolic time denotes the arterial contraction time from peak distension to the end of the cardiac cycle and includes a period when the aortic valve is closed and hence a period where there is no cardiac influence of the vascular properties of the CA.
CAA Curves and Slopes
As previously noted, changes in CAA over the cardiac cycle or distensibility have been used as a measure of arterial stiffness. Changes obtained by speckle-tracking in carotid arterial area over the cardiac cycle can therefore provide an additional method to evaluate distensibility. The software analysis package used to estimate CAS also provides the opportunity to export CA luminal area for discrete time points over the cardiac cycle. The curves also provide an opportunity to study rate of change (slopes), which should be related to the local distending pressure but in addition to the properties of the arterial wall. However, although the circumferential CAS analysis can be performed with a minimum of three carotid segments, the CAA curve requires all six segments described above. Imaging artifacts such as shadowing or anatomic variations such as short neck length contributed to impaired image quality, and as a result, a total of nine patients (six with hypertension and three with DM) were not included for this portion of the analysis, resulting in a subset consisting of 20 healthy controls, 15 subjects with hypertension, and 17 subjects with DM (52 of 61 subjects).
The CAA curve measurements were recorded with a frame rate of 78.3 ± 9.2 frames/sec, which was similar to the temporal resolution for the CAS analyses ( P = .55). Because of potential variations across multiple cardiac cycles, the CAA curves were averaged over three cardiac cycles using an in-house-developed automatic ensemble (collective) means algorithm. The ensemble-means methodology permits averaging over discrete data points recorded over multiple cardiac cycles of different lengths and varying temporal resolution. The present ensemble-means algorithm uses the QRS onset times from the three cardiac cycles to align and subsequently interpolate the CAA curves. This process results in an ensemble-means CAA curve, which is illustrated in Figure 2 for a normal control, a patient with hypertension, and a patient with DM.

From these ensemble-means curves, we calculated: (1) CAA at end vascular diastole, which identifies the beginning of arterial distention and was defined as the smallest area in the ensemble-means CAA curve ( Figure 3 ), and (2) CAA at peak of vascular systole, which was defined as the largest area in the CAA curve and marks peak distention of the CA. End of vascular diastole and peak of vascular systole were used to calculate (3) the slopes of four distinct segments of the CAA curve, which are labeled S1 to S4 and are illustrated in Figure 3 ; (4) CA distensibility, computed as (CAA at peak of vascular systole − CAA at end of vascular diastole); and (5) CA distensibility normalized to CAA at end vascular diastole, calculated as (CAA at peak of vascular systole − CAA at end of vascular diastole)/(CAA at end vascular diastole).

The slopes of segments S1 to S4 quantitatively assess the shape of the CAA curve and provide insight into the dynamics of arterial distension and arterial relaxation over the cardiac cycle. The slopes of S1 and S2 (each corresponds to 50% of the distance between end of vascular diastole and peak of vascular systole on the time axis) describe arterial distention, which is due to the distending pressure, while the slopes of S3 and S4 (again divided such that they were equally distributed on the time axis segment peak of vascular systole to end of the cardiac cycle) describe the relaxation of the CA, where diastolic blood pressure plays an important role. Figure 3 also shows a second peak/shoulder in the CAA curve. Although we did not record simultaneous arterial pulse-wave measurements in this study, the second peak/shoulder in the CAA curve could represent the dicrotic notch that is thought to be closely related to aortic valve closure. In a secondary analysis, we recalculated the slopes of S3 and S4 for two curve segments: peak of vascular systole to second peak/shoulder (S3 n ) and from second peak/shoulder to the end of the cardiac cycle in the CAA curve (S4 n ). Because the results were similar whether we used S3 and S4 or S3 n and S4 n , we present the results based on slopes S3 and S4 only.
All markers were automatically calculated with algorithms implemented in MATLAB version 7.8.0 (64-bit) (The MathWorks, Inc., Natick, MA). All calculations were performed with a 2.83-GHz 64-bit PC equipped with an Intel Quad Core Q9550 (Intel Corporation, Santa Clara, CA) processor and 8 GB random-access memory. The calculation of an ensemble-means CAA curve and the extraction of all salient markers required approximately 0.09 sec per data set.
Statistical Methods
Timing intervals were examined with and without adjustment for cardiac cycle length, because cardiac cycle length has been previously shown to have a strong correlation with left ventricular ejection time and more recently with ADPs in healthy individuals. For illustrative purposes, all slopes were multiplied by a factor of 1,000. For all analyses, significance was set at a P value of <.05. The Shapiro-Wilk test was used to assess the non-normality of continuous variables. Appropriate two-tailed parametric and nonparametric omnibus tests for comparisons among the three groups (e.g., analysis of variance and Kruskal-Wallis tests, respectively) were used to compare the participant characteristics, strain measures, time intervals, and slopes of the CAA curves. If the omnibus test proved significant, pairwise comparisons were performed between the groups using appropriate parametric and nonparametric tests (e.g., two-sample t tests and Wilcoxon’s rank-sum tests, respectively). As appropriate, Bonferroni corrections were applied to provide the “corrected P value.” Categorical tests (e.g., χ 2 and Fisher’s exact tests) were used to assess nominal variables. Differences of the CAA curves between controls and the hypertensive and diabetic group were analyzed using nonparametric Wilcoxon-Mann-Whitney tests.
Non-normal parameters, as determined by the Shapiro-Wilk test, were normalized using log or square root transformation on the basis of ladder-of-powers analyses. Pearson’s correlation coefficient ( r ) was calculated and the strength of correlations described as weak ( r < 0.40), moderate (0.40 ≤ r < 0.70), or strong ( r ≥ 0.70). Logistic regression models were used to assess the discriminative ability of the arterial stiffness markers to correctly identify subjects with diabetes and hypertension (two medical conditions that have been associated with impaired arterial function) from healthy controls. The area under the receiver operating characteristic curve (AUC) was calculated using the concordance statistic (C-statistic) of logistic regression models, and the incremental value of combining ultrasound-based markers of arterial stiffness was assessed using the AUC (C-statistic).
Results
Overall, the population ( n = 61) was middle aged (mean age, 57.5 ± 10.1 years), with 51% of the sample being women. Characteristics (other than hypertension or DM status) were similar among the three groups except for heart rate, medication use, carotid intima-media thickness, and plaque prevalence ( Table 1 ). However, heart rate for patients with hypertension was similar to that for those with DM (corrected P = .18). Individuals included in the subgroup analysis ( n = 52) for the CAA curves had similar baseline characteristics compared with the 61 subjects.
Characteristic | Controls ( n = 20) | Patients with hypertension ( n = 20) | Patients with diabetes ( n = 21) | P |
---|---|---|---|---|
Age (y) | 56.6 ± 8.4 | 58.8 ± 13.1 | 57.1 ± 8.4 | .77 |
Men | 50% | 45% | 52.4% | .89 |
Caucasian | 30% | 25% | 23.8% | .89 |
African American | 30% | 40% | 38.1% | .78 |
Hispanic | 25% | 25% | 33.3% | .79 |
Asian | 15% | 10% | 4.76% | .55 |
Systolic BP (mm Hg) | 120.8 ± 11.9 | 128.8 ± 19.4 | 127.7 ± 22.5 | .34 |
Diastolic BP (mm Hg) | 73.8 ± 7.3 | 77.9 ± 13.5 | 76.8 ± 12.0 | .49 |
Pulse pressure (mm Hg) | 47.0 ± 6.9 | 50.9 ± 11.1 | 50.9 ± 14.2 | .45 |
Heart rate (beats/min) | 63.3 ± 10.1 | 72.3 ± 12.6 | 79.0 ± 9.7 † | .0001 |
Hypertension | 0% | 100% † | 76.2% ∗ | <.0001 |
Current smoker | 0% | 2.6% | 23.8% | .08 |
Past smoker | 30% | 20% | 38.1% | .45 |
CIMT (mm) ‡ | 0.736 ± 0.122 | 0.794 ± 0.135 | 0.870 ± 0.147 ∗ | .02 |
Plaque prevalence § | 5% | 40% | 52.4% | .003 |
Amiodarone | 0% | 5% | 4.8% | <.0001 |
β-blockers | 0% | 80% | 76% | <.0001 |
Calcium channel blockers | 0% | 10% | 9.5% | <.0001 |
∗ Corrected P < .05 versus controls.
† Corrected P < .01 versus controls.
‡ CIMT for both CAs could be measured in 20 controls, 16 patients with hypertension, and 17 patients with diabetes.
§ The presence of plaque could not be evaluated in one artery (right CA) for a patient with hypertension.
CA Time Intervals
No differences were noted between patients with hypertension and those with diabetes for any comparisons related to the time intervals. The absolute arterial PDP was prolonged in patients with hypertension compared with controls for the far wall averaged CAS ( Table 2 ) but not the global net averaged CAS. For subjects with DM, the absolute ADP was shorter for both far wall and global net averaged CAS ( Table 2 ). The arterial diastolic time was significantly shorter in patients with hypertension and those with diabetes compared with healthy controls using either far wall or global average CAS. When normalized for cardiac cycle length, the PDP and peak CAS time were prolonged in patients with hypertension and those with diabetes compared with controls for both far wall and global averaged CAS, while a similar trend was seen for the ADP obtained from the global net averaged CAS curve but not for that obtained from the far wall averaged CAS curve ( Table 3 ). On the other hand, arterial diastolic time was significantly shorter in patients with diabetes and those with hypertension for global averaged CAS, while a strong trend was seen for far wall CAS.
Variables (msec) | Controls | Patients with hypertension | Patients with diabetes | P |
---|---|---|---|---|
Far wall average | ||||
PDP | 55.2 ± 22.8 | 78.3 ± 29.7 ∗ | 72.6 ± 27.8 | .006 |
Peak strain time | 328.5 ± 63.9 | 329.5 ± 66.2 | 308.6 ± 43.3 | .62 |
ADP | 273.3 ± 48.8 | 251.2 ± 54.1 | 236 ± 36.4 ∗ | .045 |
Arterial diastolic time | 648.5 ± 136.4 | 517.4 ± 106.8 ∗ | 462.1 ± 96.3 ∗ | .0001 |
Global net average | ||||
PDP | 67.3 ± 31.3 | 76.5 ± 31.4 | 72.3 ± 20.4 | .25 |
Peak strain time | 341.8 ± 68.6 | 337.4 ± 68 | 312.2 ± 39.4 | .24 |
ADP | 274.5 ± 46.7 | 260.9 ± 51.2 | 239.9 ± 33.5 ∗ | .049 |
Arterial diastolic time | 635.2 ± 113.5 | 513.1 ± 109.4 ∗ | 457.4 ± 89.8 ∗ | .0001 |
Variable | Controls | Patients with hypertension | Patients with diabetes | P | ||
---|---|---|---|---|---|---|
Model 1 | Model 2 | Model 3 | ||||
Far wall average | ||||||
PDP | 5.7 ± 2.2 | 9.6 ± 3.7 ∗ † ‡ | 9.6 ± 3.8 ∗ † | .0001 | .01 | .01 |
Peak strain time | 33.9 ± 5.3 | 39.0 ± 4.9 ∗ ‡ | 40.5 ± 6.3 ∗ † | .0009 | .02 | .02 |
ADP | 28.2 ± 3.7 | 29.4 ± 2.8 | 30.9 ± 5.1 | .09 | .19 | .19 |
Arterial diastolic time | 64.6 ± 4.9 | 60.6 ± 5.2 ∗ | 58.5 ± 7.0 ∗ | .006 | .06 | .06 |
Global net average | ||||||
PDP | 6.8 ± 2.3 | 9.3 ± 3.7 ∗ | 9.5 ± 2.8 ∗ | .0005 | .12 | .13 |
Peak strain time | 35.1 ± 3.9 | 39.9 ± 5.4 ∗ ‡ | 41.0 ± 5.6 ∗ † ‡ | .009 | .02 | .03 |
ADP | 28.3 ± 3.0 | 30.7 ± 2.9 ∗ | 31.5 ± 4.6 ∗ | .02 | .10 | .11 |
Arterial diastolic time | 64.4 ± 4.7 | 60.2 ± 6.0 ‡ | 58.6 ± 6.3 ∗ † | .005 | .04 | .048 |
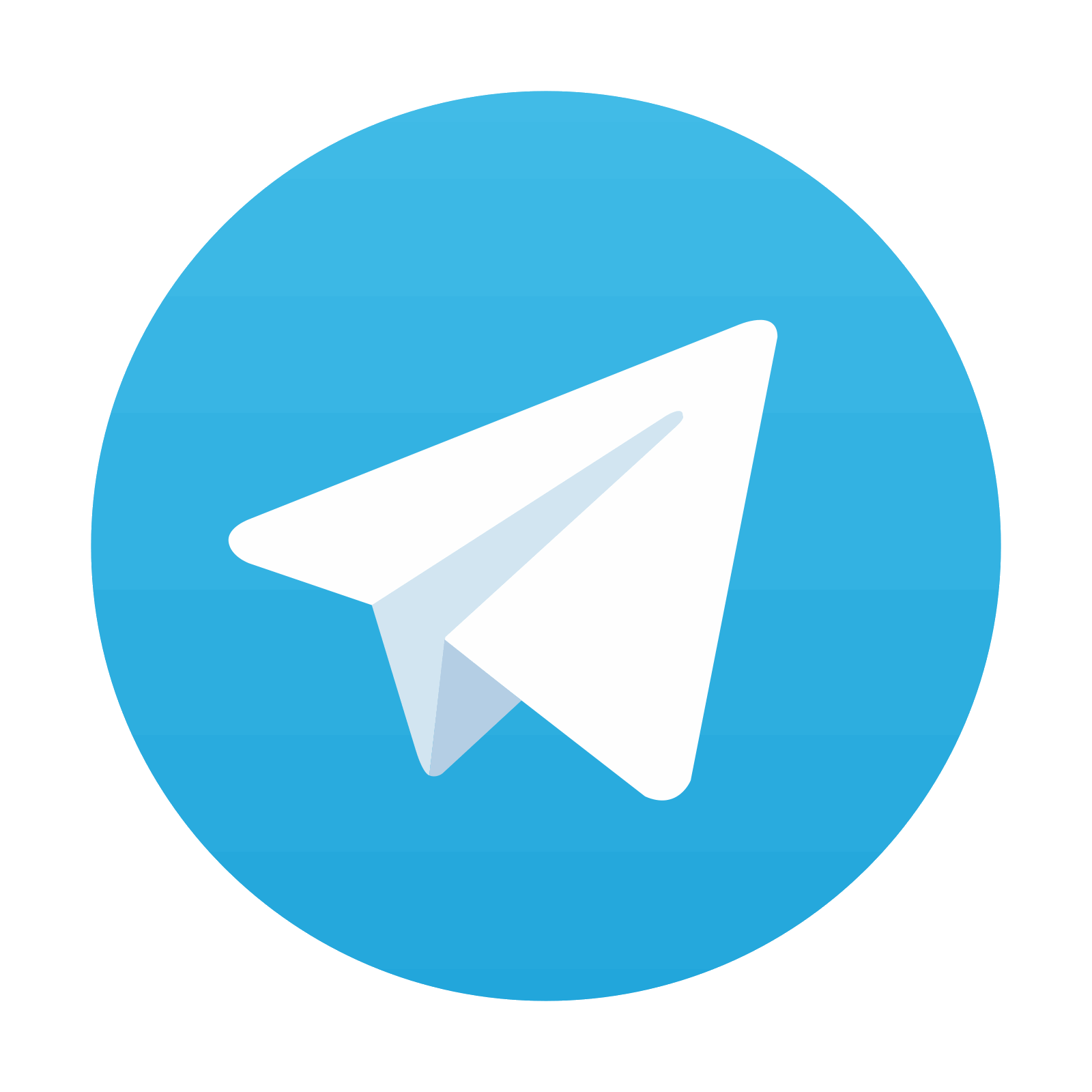
Stay updated, free articles. Join our Telegram channel
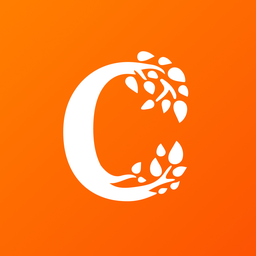
Full access? Get Clinical Tree
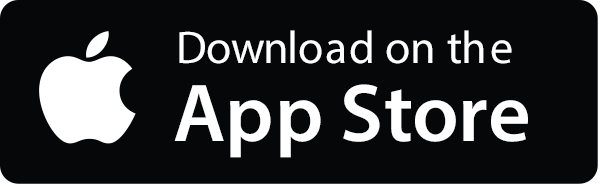
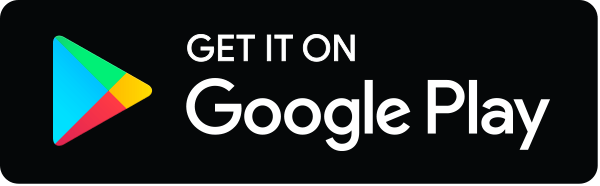
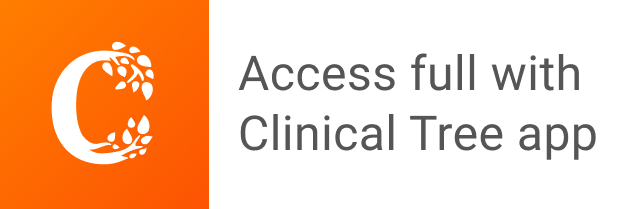