(1)
Department of Cardiovascular, Neural and Metabolic Sciences, Istituto Auxologico Italiano, Milan, Italy
All too often, myocardial ischemia is considered synonymous with coronary artery disease, as if coronary atherosclerotic phenomena were the only responsible mechanism for myocardial ischemia. On the contrary, a more correct approach to ischemic heart disease would need to consider all the factors potentially responsible for an ischemic myocardial injury, besides atherosclerotic coronary stenosis. Among them, we should consider myocardial oxygen needs, myocardial (subendocardial) flow supply and the arterial oxygen content. In order to better understand the link between arterial stiffness and myocardial ischemia, it is necessary to consider ischemic heart disease through a very broad perspective, namely, as the complex balance between subendocardial oxygen supply and demand.
In this chapter, we will analyze the role that central blood pressure plays in the ischemic heart disease, that is the relationship between aortic stiffness and myocardial ischemia. Firstly, it is important to clarify whether the association between myocardial ischemia and aortic stiffness is secondary to an independent action of aortic stiffness on myocardial supply–demand ratio, or rather whether this association depends on the presence of any factors able to affect both coronary flow and arterial wall properties. The question is, thus, how a stiff aorta might affect myocardial supply flow.
Watanabe et al. [1] described clear signs of regional myocardial dysfunction and a reduction in coronary reserve flow following a mechanical increase in aortic stiffness. These researchers reduced aortic distensibility in dogs by banding the thoracic aorta with adjustable plastic rings and studied the changes in coronary flow. Even in the absence of coronary stenosis, decreased aortic compliance was shown to be associated with inadequate subendocardial oxygenation, which could obviously be further impaired in the presence of coronary stenosis [2].
Indeed, the aorta and large arteries play a major role in the regulation of blood pressure and peripheral blood flow. It is well known that large arteries not only have a passive function in relation to the transfer of oxygenated blood from the heart to the periphery but also exert an important buffering function, as they are able to “cushion” left ventricular stroke volume thanks to their viscoelastic properties. In fact, each cardiac cycle includes a left ventricular contraction phase, in which a given amount of blood is forcibly pushed into the arterial system (systole) and a relaxation phase (diastole), in which ventricular filling occurs. Large arteries, therefore, have the task of damping the pulsatile output of the left ventricle and translating the rhythmic, intermittent, and discontinuous activity of the cardiac pump into continuous blood flow. After ejection of stroke volume and closure of the aortic valves, a great quantity of blood remains “stored” in the aorta and large arteries. The potential energy stored in the walls of the aorta in systole then turns into kinetic energy in diastole, pushing the stored blood into the bloodstream (so-called “Windkessel phenomenon”). Thus, the aorta behaves like a sort of “diastolic pump”. ensuring the achievement of a proper blood pressure level during the diastolic phase of the cardiac cycle to guarantee adequate flow to the periphery.
A number of conditions such as aging, hypertension, inflammation, media calcifications, and metabolic alterations can change the anatomical, structural, and functional properties of large arteries, thereby degrading their mechanical properties. The resulting alterations in the viscoelastic properties of the arterial system cause a stiffening in the arterial wall and a distensibility reduction in the aorta and large arteries. Under these conditions, the amount of stroke volume stored by the aorta during the systolic ejection time decreases, even drastically whereas most of the blood ejected at each systole is “pushed” directly towards the periphery of the vascular system. As a consequence, aortic systolic blood pressure increases and aortic diastolic blood pressure decreases (Fig. 6.1).


Fig. 6.1
From aortic stiffness to myocardial ischemia
The reduction in diastolic blood pressure in the aorta may cause a reduction in subendocardial blood flow supply [3]. Actually, subendocardial perfusion occurs during diastole because of the development of extravascular compressive forces through the systolic phase of the cardiac cycle. When the left ventricle contracts, the blood vessels going through the myocardial wall are compressed. The compressive force exerted on coronary blood vessels is higher in the subendocardium, where it is similar to the pressure within the left ventricle. As a result, blood flow in subendocardium is virtually withdrawn, even though subepicardial layers remain normally perfused. During the diastolic phase, the whole cardiac muscle is normally perfused again. As a consequence, the subendocardial blood flow is almost exclusively diastolic. In the absence of coronary hemodynamically significant stenosis, the diastolic pressure in coronary arteries is equal to diastolic pressure in the ascending aorta. So, not only does subendocardial coronary flow depend on diastolic time but also on aortic diastolic pressure and on the pressure gradient in diastole between coronary arteries intravascular pressure and left ventricular pressure.
Blood pressure in the ascending aorta represents the pressure against which the left ventricle has to pump during systolic contraction. If the mean arterial pressure during the systolic phase in ascending aorta is high, the left ventricle must contract more energetically to maintain adequate stroke volume. Thus, an increase in systolic blood pressure related to an increase in left ventricular afterload leads to a rise in cardiac work and consequently to an increase in left ventricular mass and myocardial oxygen needs. The development of left ventricular hypertrophy and progression towards the resulting left ventricular failure predispose to an increase in left ventricular diastolic pressure and to a prolongation in isometric contraction time, further reducing the diastolic subendocardial pressure gradient and perfusion time. The result of all these processes is a decrease in subendocardial oxygen supply.
Moreover, arterial stiffness causes an increased pulse wave velocity through the arterial system. Therefore, if the forward (centrifugal) pressure wave travels faster owing to increased arterial stiffness, similarly, the backward (centripetal) pressure wave goes back to the center at a higher speed. Under reduced arterial viscoelasticity, the earlier superimposition of the two waves, in the protomesosystolic phase of the cardiac cycle, produces a further increase in systolic blood pressure and pulse pressure values.
Another factor affected by arterial stiffness must be considered besides the increase in pulse pressure, i.e., the increase in short-term blood pressure variability. Large studies showed a link between increased arterial stiffness and an increase in short-term blood pressure variability. Among other mechanisms, such association may be partly due to a reduced sensitivity of the arterial baroreflex, i.e., of the most important mechanism for short-term control of blood pressure. This reflex system consists of stretch receptors located within the wall of the aortic arch and carotid sinus, at the origin of the internal carotid artery, connected through afferent fibers to neurons in the brain stem, from which efferent influences guarantee reflex sympathetic and parasympathetic modulation of cardiac and vascular targets. Arterial baroreceptors respond to the extent of stretching/relaxation of carotid/aortic arterial walls, rather than directly to changes in blood pressure values themselves. Baroreflex responsiveness will be reduced in individuals with increased arterial stiffness, whose vessels distend less than more elastic vessels in response to blood pressure changes. This means that the arterial baroreflex function is significantly affected by the degree of distensibility/stiffness of arterial walls, which in turn determines the degree of stretching/relaxation of carotid/aortic walls in response to blood pressure fluctuations.
As a result of the pathophysiological changes mentioned above, aortic stiffness may cause an ischemic heart injury through a reduction in subendocardial oxygen supply and/or an increase in subendocardial oxygen demand (Fig. 6.1). Aortic stiffness indeed appears to be an important factor affecting subendocardial oxygen supply–demand balance, and thus, it should be considered more than just an independent risk factor for coronary artery disease. On the other hand, ischemic heart disease should then be considered not only to be the result of a vascular atherosclerotic process affecting coronary arteries, but rather the result of a complex interaction among local coronary atherosclerotic damage, changes in vascular hemodynamics, oxygen arterial content, and the oxygen requirements of myocardial cells (Fig. 6.2). This suggests that assessment of aortic distensibility (either through magnetic resonance imaging or more simply by measuring carotid–femoral pulse wave velocity) should therefore be included among the tests recommended to screen for the risk of heart disease in daily clinical practice. In particular, on the background of these considerations, an imbalance between myocardial flow supply and demand should be always considered in the evaluation of patients with chronic myocardial ischemia.


Fig. 6.2
Factors responsible for myocardial ischemia
6.1 SubEndocardial Viability Ratio
A useful index in the assessment of cardiac ischemic risk was introduced by Gerald David Buckberg and Julien I.E. Hoffman at the beginning of the 1970s and named SubEndocardial Viability Ratio (SEVR), also known as Buckberg’s index or DPTI:SPTI ratio [4, 5]. This index reflects the subendocardial oxygen supply–demand ratio and can be defined by analyzing left ventricular and aortic pressure curves (Fig. 6.3).


Fig. 6.3
Parameters characterizing subendocardial viability ratio (SEVR). SPTI (systolic pressure–time index) reflects the cardiac workload; DPTI (diastolic pressure–time index) reflects the subendocardial flow supply. Thus, DPTI:SPTI ratio represents the subendocardial oxygen supply–demand ratio. DT, diastolic time; LVDP left ventricular diastolic pressure, LVET left ventricular ejection time, MDBP mean diastolic blood pressure, MSBP mean systolic blood pressure
The area under the left ventricular (or aortic) pressure waveform in systole (SPTI, systolic pressure–time index), from the onset of ventricular systole to the dicrotic notch, represents the left ventricular afterload and defines cardiac work. If the mean arterial pressure during the systolic phase in the ascending aorta is high, the left ventricle must contract more energetically to maintain adequate stroke volume. Thus, SPTI directly correlates with myocardial oxygen needs and mainly depends on left ventricular ejection time, ejection pressure, and myocardial contractility.
The area between the aortic and left ventricular pressure curves in diastole represents the pressure that affects the coronary blood flow and maintains adequate subendocardial blood supply in the diastolic phase of the cardiac cycle (DPTI, diastolic pressure–time index). During the systolic phase, blood supply to the subendocardial layers is not allowed, owing to the presence of two extravascular compressive forces: (1) the first force is left ventricular intracavitary pressure, which is fully transmitted to the subendocardial layers, but which falls off to almost zero at the epicardium and (2) the second one is the force developed during left ventricular contraction itself leading to coronary vascular occlusion. The overall compressive force exerted on coronary blood vessels is higher in the subendocardium, whereas it is similar to the pressure within the left ventricle. Thus, blood flow to subendocardial fiber layers is virtually absent in systole, even though subepicardial layers remain normally perfused. Along this line, experimental studies clearly showed that a reduction in aortic distensibility alters the transmural myocardial blood flow distribution of the left ventricle and decreases the subendocardial–subepicardial flow ratio. During the diastolic phase, the degree of myocardial perfusion largely depends on DPTI, which, in turn, is a function of the coronary arterial diastolic pressure, the pressure gradient in diastole between coronary arteries and left ventricular pressure, and the duration of diastole. DPTI is obtained by subtracting the area relative to left ventricular mean diastolic blood pressure (LVDP) from the area, in diastole, of the aortic pressure curve. LVDP can be, substantially and successfully, replaced by an estimate of the left ventricular (or atrial) end-diastolic pressure, which is more easily assessed, non-invasively by echocardiography.
A very easy method for the numerical calculation of left ventricular end-diastolic pressure (LVDP) based on the estimation of mean arterial pressure (MAP) and ejection fraction (EF) has been proposed by Abd-El-Aziz:


Therefore, the subendocardial viability ratio can be calculated using the following formula:


The diastolic pressure–time index (DPTI) is given by the area between the aortic and left ventricular pressure curves in diastole:
where AoMDBP represents the aortic mean blood pressure in diastolic phase, while LVDP represents the left ventricular mean diastolic pressure and DT represents the diastolic time.

Systolic pressure–time index (SPTI) represents the area under the aortic pressure curve in systole:
where AoMSBP represents the aortic (=left ventricular) mean systolic blood pressure and LVET represents the left ventricular ejection time, so:
where MDBP and MSBP represent the mean value of central (aortic, carotid) blood pressure during the diastolic and systolic phase of the cardiac cycle respectively. The left ventricular diastolic pressure (LVDP) can be defined by means of echocardiography, assessing the left ventricular end-diastolic pressure.


To sum up, the subendocardial viability ratio describes the relationship between supply and demand, i.e., between the myocardial blood supply and myocardial oxygen requirement.
The DPTI:SPTI ratio represents, thus, the balance between oxygen subendocardial supply and demand. In the elderly and in the presence of aortic stiffness, these two parameters significantly change (SPTI increases and DPTI decreases) and the subendocardial oxygen supply–demand ratio (DPTI:SPTI ratio) is greatly reduced, as clearly shown in Fig. 6.4, a finding which highlights the possible usefulness of subendocardial oxygen supply–demand ratio assessment in clinical practice. However, in the past, an invasive arterial catheterization was needed to assess it, which was its major limitation for it to be applied in a clinical setting.


Fig. 6.4
Systolic pressure–time index (SPTI) and diastolic pressure–time index (DPTI) (a) in a young healthy adult with normal viscoelastic properties of aorta and (b) in an old hypertensive patient with heart failure and increased aortic stiffness. SPTI reflects the cardiac workload; DPTI reflects the subendocardial flow supply. Thus, DPTI:SPTI ratio represents the subendocardial oxygen supply–demand ratio. Lower panels show the corresponding subendocardial flow velocity in both the conditions. Several studies have stressed the similarity between the morphology of the diastolic central pressure waveform and the morphology of the coronary blood flow curve. DT diastolic time, LVET left ventricular ejection time, ICT isovolumic contraction time
The Rotterdam Study demonstrated that an increase in pulse wave velocity (PWV) is accompanied by an increase in SPTI and by a decrease in DPTI, and, therefore, by a significant reduction in SEVR. The boost in oxygen consumption, generated by the increase in afterload owing to arterial stiffness, and the reduction in oxygen supply to the myocardium are elements which can help us understand the association between aortic stiffness (as shown by PWV) and cardiovascular mortality and morbidity.
6.1.1 Reference Values for SEVR
Are there any normal values for SEVR? What are the reference values for this parameter?
A “critical” value has been proved for SEVR; it corresponds to 0.45 (i.e. 45 %), below which the ratio between subendocardial flow and subepicardial flow, per gram, is reduced in the left ventricle as a signal of insufficient subendocardial vascularization [6]. However, even thanks to the coronary autoregulation, over this critical value, a linear relationship between SEVR and coronary blood flow does not exist.
In other words, for values greater than 0.45 (i.e. when DPTI is more than 45 % of SPTI), the degree of subendocardial vascularization remains almost constant (Fig. 6.5a) and the ratio between subendocardial flow and subepicardial flow, per gram, remains within the reference range.


Fig. 6.5
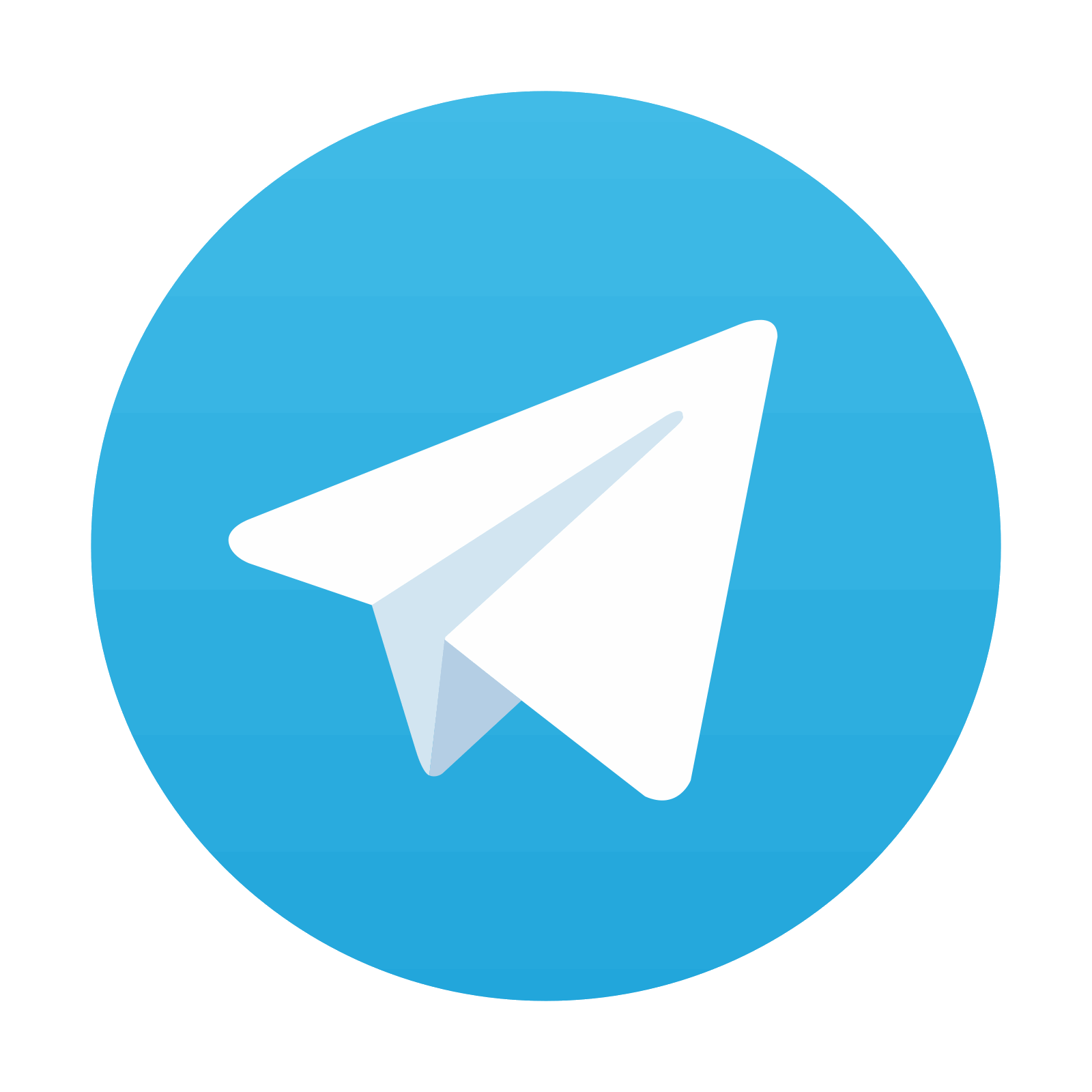
(a) Flow ratio per gram of left ventricular subendocardial to subepicardial muscle with respect to subendocardial viability ratio (SEVR = DPTI:SPTI). The white dots indicate patients with anemia; dark dots indicate control subjects or patients without anemia. (b) Flow ratio per gram of left ventricular subendocardial to subepicardial muscle with respect to subendocardial viability index corrected for oxygen content [8]. The figure highlights that oxygen content should be taken into account in the assessment of subendocardial viability. DPTI diastolic pressure–time index, SPTI systolic pressure–time index. Normally, flow per gram per minute is about 1–1.2 times higher in the subendocardium than in the subepicardium. Subendocardial muscle becomes ischemic when the proportion of total coronary blood flow delivered to the subendocardial region becomes reduced (subendocardial/subepicardial flow ratio <0.8). Flow ratios below 0.8 always occurs whenever DPTI/SPTI is less than 0.45 or CaO2 × DPTI/SPTI is less than 9
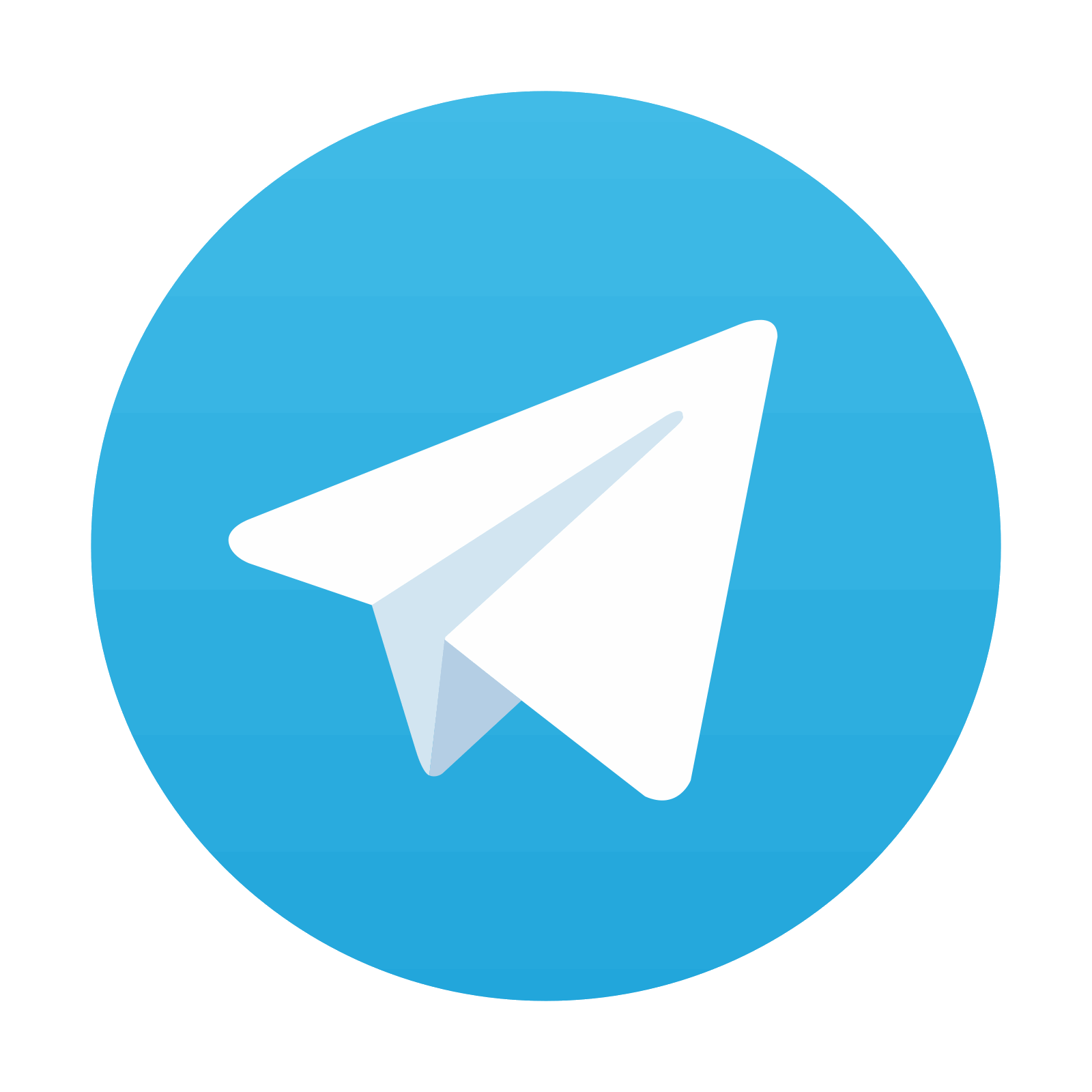
Stay updated, free articles. Join our Telegram channel
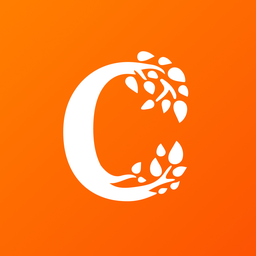
Full access? Get Clinical Tree
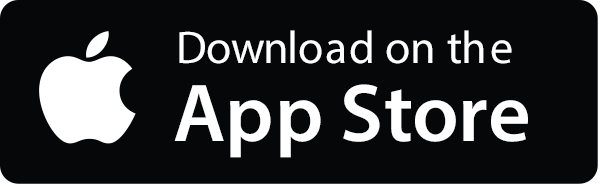
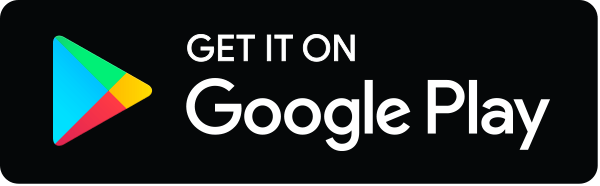
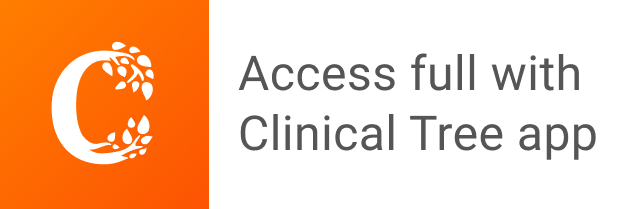