Fig. 16.1
Types of aneurysm; a and b fusiform aneurysms; c and d saccular or berry aneurysms
Factors leading to susceptibility for aneurysm formation include both genetic and environmental factors. Hereditary conditions such as Marfan’s and Ehlers-Danlos syndromes are associated with collagen disorders leading to arterial wall weakening and both these syndromes have a predisposition to aneurysms. Environmental risk factors include hypertension, cigarette smoking, family history of aneurysmal disease and oestrogen deficiency in the postmenopausal female (Mehra et al. 2011, Lasheras 2007). These risk factors translate into biological processes in the arterial wall. Development and growth of an aneurysm is an interplay between these biological processes and the mechanical environment.
Development and growth of aneurysms is associated with weakening of the wall through loss of elastin with subsequent remodelling of the wall in an attempt to renormalise forces on and within the wall. The interplay between the local mechanical environment and the local biology is thought to be paramount in the initiation and growth of aneurysms, but the exact details of this relationship remain the subject of research. Once established, the aneurysm continues to grow and for some aneurysms there comes a point when the stress within the wall exceeds the wall strength and the aneurysm will rupture. Most aneurysms give no symptoms prior to rupture. The subsequent bleeding into the surrounding tissues is associated with a high mortality rate; 90 % for abdominal aortic aneurysm (AAA) rupture and 45 % for cerebral aneurysm rupture.
This chapter will concentrate on the 2 most common types of aneurysm; the abdominal aortic aneurysm and the saccular cerebral aneurysm. Further reading is provided by Lasheras (2007), Sforza et al. (2009), Humphrey and Taylor (2008), Humphrey and Holzapfel (2012), Vorp (2007), McGloughlin and Doyle (2010), Penn et al. (2011), Wong and Poon (2011) and Meng et al. (2014).
16.1.2 Aneurysms and the Law of Laplace
A simple understanding of aneurysm mechanics involves the Law of Laplace which was introduced in Chap. 5. Equations 16.1 and 16.2 describe the tension in the wall of a thin-walled cylinder and a thin-walled sphere respectively. In each case the tension T increases with diameter d.



(16.1)

(16.2)
In biomechanics it is more common to use circumferential stress H rather than tension. Equations 16.3 and 16.4 are for a thin-walled cylinder and a thin-walled sphere respectively, where w is wall thickness.



(16.3)

(16.4)
An artery may be considered as a thin-walled cylinder, a saccular aneurysm resembles a sphere and a fusiform aneurysm has a shape between a cylinder and a sphere. These equations suggest that the circumferential stress, and hence the risk of rupture, increases with diameter. This helps understand why diameter is the current clinical method for evaluating risk of rupture in both abdominal aortic aneurysm and in saccular CAs.
In practice there are limitations to this simple model. Arteries are not just pipes and will remodel in an attempt to normalise stresses. In practice this means that there will be changes in wall thickness as the aneurysm grows. Rupture represents mechanical failure of the tissues where circumferential stress exceeds tissue strength. The tissue strength will depend on the local tissue composition which will vary from point to point around the wall. Aneurysms have a complex geometry which does not correspond to either a cylinder or a sphere. In evaluation of rupture risk all these factors need to be accounted for, which is the aim of patient specific modelling as described in Chap. 14.
16.2 Cerebral Aneurysms
16.2.1 Cerebral Aneurysm Disease
Cerebral aneurysms are localised increases in diameter of arteries supplying the brain. These are also referred to as intracranial aneurysms. Some 4–6 % of the population aged greater than 30 years have a CA (Wardlaw and White 2000). The main clinical complication is rupture leading to bleeding. The blood accumulates beneath the arachnoid membrane which surrounds the brain, producing a subarachnoid haemorrhage. The risk of rupture of a CA is low at 0.1–1 % per year (Juvela 2004). However the consequences of rupture are severe for the patient. Mortality following aneurysm rupture is 45 %, 30 % of survivors demonstrate moderate to severe disability, and the remainder have increased risk of re-bleeds and stroke (Brisman et al. 2006). Most CAs are asymptomatic so that the first knowledge of the presence of a CA is the symptoms associated with subarachnoid haemorrhage (‘thunderclap headache’, nausea, vomiting, altered consciousness of varying degree from drowsiness to complete loss of consciousness, and disturbance of vision). In some cases larger CAs may themselves produce similar symptoms immediately prior to rupture. Treatment of CAs involves a variety of procedures concerned with sealing off the aneurysm and these are considered further in Sect. 16.5.
CAs are of 2 main types, saccular and fusiform. Saccular aneurysms are round in shape and also referred to as ‘berry aneurysms’; these usually occur at the branching point of arteries of the Circle of Willis and are the most common type of CA (80–90 %). Fusiform aneurysms involve an overall widening of the artery and usually do not rupture. Saccular aneurysms are also classified by size; small (<15 mm diameter), large (15–25 mm), giant (25–50 mm) and super-giant (>50 mm). The remainder of Sect. 16.2 will be concerned with saccular CAs.
16.2.2 Haemodynamics
Saccular CAs are not conduit vessels; blood flow enters and exits the aneurysm via the neck. The flow pattern within the aneurysm depends on its orientation with respect to the parent artery (Moftakher et al. 2007) (Fig. 16.2). Figure 16.2a shows a sidewall aneurysm. Flow in the aneurysm recirculates, often through the entire cardiac cycle. Blood velocity is very low, wall shear stress is very low and there is low pressure on the dome (far wall) of the aneurysm. In the case of a bifurcation aneurysm 2 flow patterns are seen. The most common is illustrated in Fig. 16.2b. Here flow enters the aneurysm from the parent artery through a channel within the aneurysm neck, circulates around the aneurysm, and exits through a separate channel in the neck. Velocities, wall shear and pressure at the dome are low. The second flow pattern seen in bifurcation aneurysms is illustrated in Fig. 16.2c. Here flow from the parent artery enters the aneurysm as a jet which impacts on the dome, flow then exits in a random manner through the neck. The impact of the jet causes local high wall shear and pressure on the dome. Because of the persistent flow through the cardiac cycle most CAs have no thrombus, as opposed to AAAs where thrombus is common.


Fig. 16.2
Schematic of flow within saccular cerebral aneurysms, with indications of blood velocity, dome pressure gradient (with respect to the neck) and wall shear stress. a Recirculation recirculating flow in a side-aneurysm. b Jet impact flow from the parent artery forms a jet which impacts on the aneurysm wall. c Circulating flow flow from the parent artery enters the aneurysm through a channel in the neck, circulates as a result of the geometry of the aneurysm, and exits through a separate channel in the neck
16.2.3 Initiation, Growth and Rupture
The composition of cerebral arteries is different to that of systemic arteries. Cerebral arteries possess much less elastin in the medial layer, the external elastic lamina is absent, and there are structural abnormalities at the apex of bifurcations, all of which are thought to make cerebral arteries prone to saccular aneurysms. Cerebral aneurysms are characterised by loss of elastin and smooth muscle cells with consequent thinning of the medial layer (Frösen et al. 2012). Collagen fibres remodel, probably in an attempt to mechanically stabilise the aneurysm. The result is an increase in wall stiffness, with the main load-bearing performed by the adventitia rather than the media.
Initiation, growth and rupture of the CA are governed by an interplay between the mechanical environment and the local biology. The exact details of this remain the subject of research for CAs, though central to this will be presence of an intact endothelial layer. Unruptured aneurysms lack an endothelial layer (Frösen et al. 2004), and prior to this there is endothelial dysfunction. An intact endothelial layer is essential for an artery to adapt to its mechanical environment. This suggests that as the CA evolves, initially the endothelial layer is intact and the aneurysm will remodel in an attempt to normalise its mechanical environment. Later there is endothelial dysfunction with impairment in the aneurysms ability to maintain the mechanical environment. Finally there is loss of the endothelial layer and the aneurysm’s ability to remodel is largely absent. Reviews of this area are provided by Sforza et al. (2009), Meng et al. (2014), Penn et al. (2011) and Selimovic et al. (2013) from which the analysis below is derived. The latter paper (Selimovic) describes computational models of aneurysm growth.
16.2.3.1 Initiation of Saccular CAs
High wall shear stress hypothesis. Meng et al. (2014) proposed a high WSS hypothesis discussed here. At a bifurcation, flow from the parent artery impacts on the bifurcation apex producing high WSS and also a positive WSS gradient along the flow. The endothelium detects these WSS abnormalities, and a series of biological events occurs resulting in degradation of elastin, medial thinning and formation of a bulge, which constitutes the early aneurysm.
Low wall shear stress hypothesis. Meng et al. (2014) also proposed a low WSS hypothesis discussed in this section. In the paper on computational modelling of aneurysm growth, Selimovic et al. (2013) report that when elastin degradation was associated with high WSS this led to aneurysm formation, but of the fusiform type rather than the saccular type. Linking low WSS to elastin degradation resulted in formation of a saccular aneurysm. Selimovic et al. note that a possible mechanism for low-WSS initiation is the release of matrix-metalloproteinases (MMPs) by inflammatory cells, which degrade elastin.
Flow instability hypothesis. In their study on computational modelling of aneurysms Selimovic et al. note that it may actually not be the magnitude of the WSS which is important, but the nature of the flow including flow oscillation and instability.
16.2.3.2 Growth of Saccular CAs
High wall shear stress hypothesis. In an established aneurysm with impact of a jet on the dome (Fig. 16.2c), there will be a region of high WSS. This high WSS is detected by the endothelium, which in the early stages of aneurysm development is still intact, leading to release of MMPs and matrix degradation. There may be a local bleb formed at the region of impact. Remodelling of collagen stablises the aneurysm.
Low wall shear stress hypothesis. In an established aneurysm where there is recirculating flow (Fig. 16.2b) the wall shear stress will be low. This low WSS is sensed by the endothelium, which in the early stages of aneurysm development, is still intact. This also leads to release of MMPS, but by an inflammatory route, which also leads to matrix degradation.
16.2.3.3 Rupture of Saccular CAs
A possible sequence of events concerning rupture is described here. Rupture occurs when the circumferential stress exceeds the local wall strength. Wall shear stress itself is too small a force to cause rupture. However the growth of the aneurysm is effected by WSS, causing changes to the wall which leave the aneurysm vulnerable to rupture. Following on from the above sub-section, where there is loss of matrix material, the aneurysm remodels in an attempt to achieve mechanical stability. This process must rely on an intact endothelium in order to drive remodelling. It is however noted above that aneurysms are commonly associated with loss of endothelium which must mean that at some point the remodelling apparatus is also lost. With loss of endothelium driven remodelling the aneurysm is unable to mechanically stabilise itself and after this point the aneurysm is at risk of rupture.
16.2.4 In Vivo Imaging and Patient Specific Modelling
Traditional visualisation of CAs was undertaken using angiography which involved an arterial puncture. A number of non-invasive techniques have been used to diagnose the presence of CAs including CT angiography (CTA), rotational angiography (RA) and magnetic resonance angiography (MRA). All these techniques provide 3D data from which measurements may be made of aneurysm dimensions. In addition the 3D geometries may be used to derive indices describing shape and as the input to patient specific modelling. Ultrasound systems may also be used for visualisation of CAs. Further reading on the imaging of cerebral aneurysm is provided in Hoskins et al. (2011).
16.2.4.1 Measurements of Size
The measurements commonly made on a saccular aneurysm are illustrated in Fig. 16.3. The diameter of a saccular aneurysm is measured as the largest of the height (H), and the 2 orthogonal widths. As noted above the aneurysm is classified according to diameter (small <15 mm diameter, large 15–25 mm, giant 25–50 mm, super-giant >50 mm); and risk of rupture increase with diameter (Wermer et al. 2007).


Fig. 16.3
Definition of dimensions of a saccular aneurysm measured from medical imaging data
Aneurysms with a higher aspect ratio are at greater risk of rupture (Dhar et al. 2008; Ujiie et al. Ujiie et al. 1999; Weir et al. 2003; Nader-Sepahi et al. 2004), so this measurement may be used for clinical evaluation. The aspect ratio may also be used to help plan therapy; a ratio of >2 is considered as favourable for coil occlusion (Meyers et al. 2009). Measurement of aneurysm volume helps define the size of coil or balloon needed in therapeutic treatment. More complex shape descriptors involving the whole 3D geometry have been developed in research studies, whose details are outside the scope of this book (Millán et al. 2007).
16.2.4.2 Pulsatility
The aneurysm will change volume during the cardiac cycle as a result of the change in blood pressure. Dynamic CTA has been used to measure this change in volume (Hayakawa et al. 2005; Ishida et al. 2005; Krings et al. 2009). Ishida et al. (2005) noted pulsation in 9 of 28 saccular aneurysms and in 3 of 5 non-saccular aneurysms. Hayakawa et al. (2005) investigated 23 patients with ruptured aneurysms, of which 4 showed pulsation whose location corresponded to the site of rupture in all 4 cases. It was hypothesised in these studies that the pulsation occurred where the wall was thin, and that this was a site at risk of rupture.
16.2.4.3 Haemodynamics and Wall Stress
MRI may be used to measure blood velocities from which 3D data on haemodynamic flow patterns and wall shear stress may be calculated (Moftakhar et al. 2007). Haemodynamics may also be obtained as the output from patient specific modelling (see Chap. 11). Figure 16.4 shows examples of flow streamlines and wall shear stress in a saccular aneurysm. Patient specific modelling may also be used to estimate the distribution of circumferential wall stress (Fig. 16.5).



Fig. 16.4
Haemodynamics in a Basilar tip aneurysm with two lobes calculated from patient specific modelling. The basilar artery enters from the bottom of the image and is nearly vertical. a Flow streamlines; the velocity range is 0–2 ms−1. b Wall shear stress; the range is 0–50 Pa. Images kindly provided by Dr. Arjan Geers (Edinburgh University). In both figures, red is ‘high’ and blue is ‘low’ ends of the range

Fig. 16.5
von Mises stress (Pa) in cerebral aneurysms calculated from patient specific modelling. Image kindly provided by Dr. Noel Conlisk (Edinburgh University)
16.2.5 Treatment
This section presents a very brief description of the treatment of CAs. Further reading on diagnostic and treatment pathways are provided in Wardlaw and White (2000) and Mehrar et al. (2011). Most CAs that rupture are undiagnosed at the time of rupture and present as an emergency. Treatment of the ruptured aneurysm involves exclusion of the aneurysm from the circulation. The decision to treat the unruptured aneurysm is based on aneurysm diameter. Treatment is considered if the diameter is greater than 7 mm, however if there are other risk factors then treatment is considered if the diameter is greater than 3 mm diameter. Historically surgical clipping of the aneurysm was the preferred method for treatment of a cerebral aneurysm. This is highly invasive involving open surgery with placement of a clip across the aneurysm neck to separate the aneurysm from the main arterial flow channel. It is much more common to perform intervention by catheter based techniques under fluoroscopic guidance (White et al. 2015). The most common technique is the insertion of coils in the aneurysm which are thrombogenic, so that the aneurysm is sealed off by the presence of thrombus. Increasingly coiling is combined with the placement of a stent. The stent helps prevent ingress of the coil into the main arterial channel, hence allowing more aggressive coil placement, and provides a mesh on which an endothelium can grow. Other devices and methods which are used in interventional treatment include balloon remodelling, flow diverters and intrasaccular flow disruptors.
16.3 Abdominal Aortic Aneurysms
16.3.1 Abdominal Aortic Aneurysm Disease
As the name implies, AAAs are localised increases in the diameter of the abdominal aorta (see Fig. 16.6), however they can often extend distally beyond the iliac bifurcation, or begin above the abdominal aorta. They are defined as a maximum aortic diameter ≥3.0 cm with the un-diseased diameter being around 2 cm. The incidence rates of AAAs range from 2 to 8 % for men over 65 years and the prevalence is 4 times lower in women. Over recent years, the incidence rates appear to be falling, with this being attributed to the change in smoking habits in developed countries (Lederle 2011).


Fig. 16.6
CT reconstruction of an abdominal aortic aneurysm
Most AAAs are asymptomatic so that the first time the patient is aware of an aneurysm is after rupture. The most common symptom is sudden pain in the abdomen; other symptoms include low blood pressure, loss of consciousness and a pulsatile mass in the abdomen. Following rupture there is usually considerable internal bleeding and this is the main cause of death. If an unruptured AAA is detected, usually with medical imaging, the primary criterion determining surgical intervention is the size of the AAA, more specifically, the maximum diameter. If the maximum anterior-posterior diameter of the AAA exceeds 5.5 cm in men or 5.0 cm in women, and the patient is fit for surgery, repair is offered. Risk of rupture increases with maximum AAA diameter. The annual rupture risk increases from 0.5 to 5 % for diameter of 4.0–4.9 cm, 3–5 % for 5.0–5.9 cm, 10–20 % for 6.0–6.9 cm, and 20–40 % for 7.0–7.9 cm (Brewster et al. 2003).
A minority of AAA are symptomatic in that the patient develops a variety of symptoms associated with imminent rupture. These symptoms include abdominal pain, lower back pain and a pulsatile abdominal mass. Generally symptomatic patients are offered urgent repair of their AAA (De Martino et al. 2010).
16.3.2 Mechanics and Haemodynamics
The healthy aorta is often approximated as a cylinder; however, AAAs can develop into highly irregular shapes with regions of high curvature and highly tortuous centrelines. As such, the mechanics used to determine the stresses and strains cannot be approximated using the Law of Laplace (see Chap. 5.1.5). It is these stresses and strains that contribute to the growth, remodelling and eventual rupture of the AAA, hence the large research focus aimed at accurately predicting them (see Chap. 11.4.3). A material will fail when the local stress exceeds the local strength, and although there are many biological processes also at play, this general understanding also applies to AAA. Using patient specific modelling (PSM—discussed in detail in Chap. 11), it is possible to estimate the stress acting on the AAA wall in vivo (Fillinger et al. 2002, 2003; Truijers et al. 2007; Doyle et al. 2009; Gasser et al. 2010; Doyle et al. 2014). By coupling this data with knowledge of the AAA wall strength (Thubrikar et al. 2001; Raghavan et al. 2011, 2006), it is possible to determine the likelihood of rupture. However, predicting in vivo wall strength remains a significant challenge (vande Geest et al. 2006; Reeps et al. 2013).
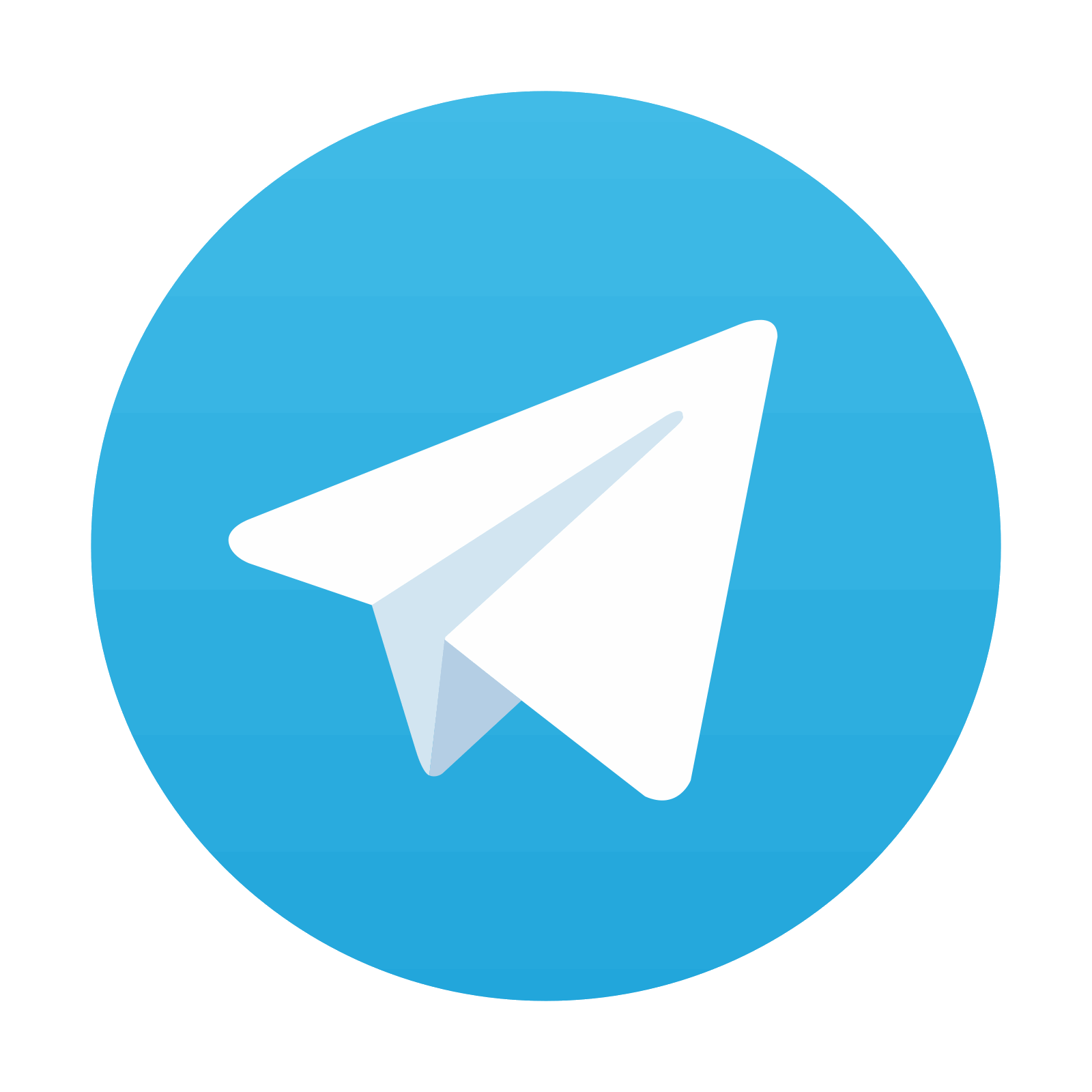
Stay updated, free articles. Join our Telegram channel
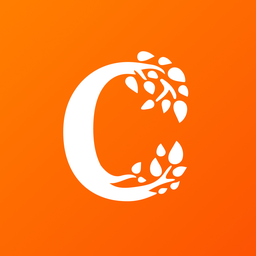
Full access? Get Clinical Tree
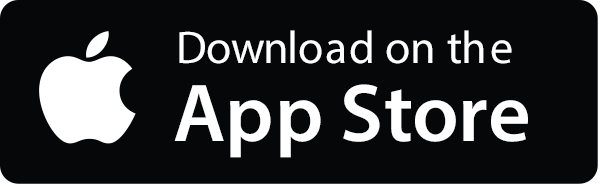
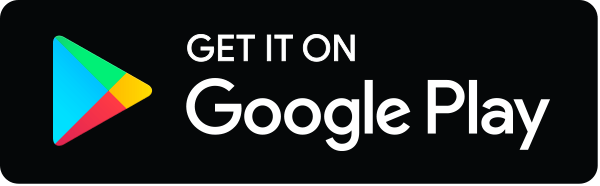