INTRODUCTION TO ALVEOLAR GAS DIFFUSION
Learning Objectives
The student will be able to define the terms governing alveolar O2 diffusion, and the circumstances when O2 uptake is limited by perfusion or diffusion.
The student will be able to calculate the percentage of Q̇ comprising physiological shunt by using appropriate patient data and knowledge of HbO2 interactions in blood.
The student will be able to summarize processes in CO2 excretion and the manner by which blood CO2 acts to maintain normal blood pH.
The student will be able to use patient data to distinguish among respiratory acidosis, metabolic acidosis, respiratory alkalosis, and metabolic alkalosis.
Once gases reach the alveolar parenchyma by ventilation, absorption into the blood or excretion from it occurs not by convection, but by molecular diffusion according to a physiological restatement of Ohm’s law (Fig. 9.1). Diffusion of any gas through the septal barrier and into the blood is proportional to the alveolar epithelial surface area (SA) and the alveolar capillary endothelial surface area (SC) comprising the membrane available for such exchange. Quantitative measurements on electron photomicrographs of normal lung have estimated SA and SC to each be 50-70 m2. This symmetry of SA and SC dimensions is perhaps not surprising, given the importance placed earlier on good V̇A/Q̇ matching. Alveolar diffusion of any gas is inversely proportional to septal barrier thickness, estimated as its harmonic mean (τS) to emphasize statistically the thinnest regions where diffusion is presumably favored.
FIGURE 9.1
Alveolar diffusion as described by a physiological version of Ohm’s law: Flow = (P1 − P2)/R, where P1 and P2 are the “source” and “sink” gas partial pressures in two adjacent compartments. Conductance (C) is the inverse of resistance R, and thus Flow = C • (P1 − P2). C is proportional to the average of all alveolar and capillary surface areas [(SA + SC)/2] in the septa available for diffusion, and inversely proportional to the harmonic mean thickness of those septal barriers (τs). C is also proportional to Krogh’s coefficient of diffusivity (D) for each gas, which is in turn the ratio of its solubility in saline divided by the molecular weight (MW) of each gas.
Given these lung anatomical features that affect all gases, other factors will determine whether diffusional equilibrium is achieved between an alveolar airspace and the blood flowing through its capillaries. Again by analogy to Ohm’s law, each gas diffuses proportionally to the pressure gradient between its “source” (P1, the higher value) and its “sink” (P2, the lower value). For O2, P1 = PAo2 (Chap. 8) and P2 = PV̄o2 as measured by a pulmonary artery catheter, yielding a normal inwardly directed gradient of ~60 mm Hg on room air. That gradient is dramatically affected by both FIo2 and PB insofar as those affect PIo2 and thus PAo2. For CO2, P1 = PV̄co2, and P2 = PAco2 (= Paco2), for a normal outwardly directed gradient of 5-6 mm Hg and one that is less sensitive to FIo2 or PB than that for Po2. Indeed, data in Table 3.2 were used to make this point earlier of a tenfold larger gradient for O2 despite that the flow of CO2 outward and O2 inward are nearly the same. How can this occur? Gases will diffuse at different rates, despite equal values for SA, SC, τS, and (P1-P2), because of differing coefficients of diffusivity (D), defined as the solubility of a gas in aqueous media divided by its mass-dependent rate of gaseous-free diffusion. The value of D for CO2 is ~20 times that of O2 in biological systems, implying that lung disorders will present more often with hypoxemia (low blood O2 content) than with hypercarbia (high blood CO2 content). One can also appreciate that alveolar diffusion of all gases will diminish in a disease like emphysema where SA and SC are decreased (Fig. 9.2). Likewise, alveolar diffusion decreases in those diseases for which τS is increased, for example during the edema of acute lung injury (Chap. 28) or after the development of fibrosis in some interstitial lung diseases that show a restrictive pattern by pulmonary function test (PFT) (Chap. 24).
FIGURE 9.2
SA is a key determinant of diffusion, and any decrease reduces maximal O2 uptake and CO2 excretion. Three images of lung tissue (all at the same magnification) are shown with their Emphysema Severity Scores assigned by the American Thoracic Society. A score of 0-2 would be normal for urban adults. From Nagai et al. Am Rev Respir Dis. 1989;139:313-319.
DISTINGUISHING DIFFUSIONAL AND PERFUSIONAL LIMITS TO GAS EXCHANGE
Given this discussion, what time constraints exist on gas diffusion in the lung? This question can be answered by considering capillary transit times of erythrocytes in the lung in relation to the equilibration rate of alveolar gases with the interiors of those red blood cells (RBCs) (Fig. 9.3). An RBC spends ~0.8 seconds in an alveolar capillary at a resting Q̇ but less than 0.3 seconds when Q̇ approaches maximal values. The long RBC transit time at low Q̇ is usually sufficient for N2O (an anesthetic gas), CO, CO2, and O2 to equilibrate across the alveolar-capillary barrier, that is, P1 ≅ P2 for these gases near the venular end of alveolar capillaries. By example, N2O is very soluble in blood but does not bind to Hb. Thus, it equilibrates across the barrier regardless of the original (PAn2o-Pc’n2o) or transit time, so that its absorption is perfusion limited only by Q̇. If Q̇ increases, then N2O uptake increases because more RBCs pass through the alveolar capillaries in the same interval of time. In contrast, CO binds to Hb in such large amounts that no RBC spends enough time in an alveolar capillary to equilibrate PAco with Pc’co. Thus, the absorption of CO is diffusion limited, and simply increasing Q̇ will not necessarily increase CO uptake. Rather, the alveolar uptake of CO and other diffusion limited gases will more likely increase if SA and SC increase, or if τs decreases.
FIGURE 9.3
Equilibration rates of CO, nitrous oxide (N2O), and O2 along the alveolar capillary. Pcn2o equilibrates rapidly with alveolar PAN2O, well before the capillary ends, and so N2O is perfusion limited. In contrast, PĆco in capillary blood is nearly unchanged during one capillary transit, and thus CO transfer is diffusion limited. O2 transfer into alveolar blood can be perfusion limited or diffusion limited, depending upon conditions. From West, Respiratory Physiology; 2005.
Normally O2 has an intermediate equilibration rate to those of N2O or CO. In healthy individuals, O2 is a perfusion-limited gas over a wide range of Q̇ (Fig. 9.3), and at sea level alveolar O2 uptake usually is not considered to be the rate-limiting step to maximal O2 consumption, V̇o2max (Chap. 12). However, in diseased lungs with destroyed or fibrotic alveolar septa, O2 becomes diffusion limited during moderate exercise, and potentially at rest despite long RBC transit times. Even in healthy individuals, O2 becomes a diffusion-limited gas when PAo2 is reduced by a low FIo2 or by the reduced PB at high altitude (Chap. 13).
Based on its greater aqueous solubility, CO2 diffuses into and out of tissues ~20 times faster than O2 per mm Hg of pressure gradient. Consequently, there is usually ample capillary transit time for CO2 to achieve equilibrium despite its much smaller partial pressure gradient versus O2. However, under conditions of reduced RBC transit time or with thickening of the alveolar septal membranes, CO2 transfer may be incomplete and result in hypercarbia.
ESTIMATING MAXIMAL PULMONARY DIFFUSING CAPACITY
The long equilibration time of inhaled CO is used clinically to estimate the single-breath lung diffusing capacity for CO (DLCO). As detailed in Chap. 16, this pulmonary function test is most often conducted on patients who are suspected of having reduced SA or SC, or increased τs. Subjects begin the maneuver at their RV and inhale gas with a low FIco (usually ~0.003) up to their TLC. Once at TLC, the breath is held for 10 seconds and then exhaled completely. Concentrations of CO in the exhaled gas are measured to establish FĒco and FÉco (= FAco), and thus PAco by Dalton’s law. These data yield the CO uptake rate (V̇co) plus the “P1” from the equation in Fig. 9.1 that drove its absorption. “P2” in that same equation, being the subject’s PĆco, is considered zero since inhaled CO never equilibrates with blood in the capillaries. The diffusivity coefficient DCO is constant because the aqueous solubility and mass of CO are known. DLCO is computed then as:
where carbon monoxide conductance, CCO = [DCO · (SA + SC)]/(2 · τs). Thus, the working equation for the DLCO test becomes:
Since all terms on the right side of this equation are measured by the single-breath DLCO procedure, the test yields a robust “lump sum” or aggregate estimate of a subject’s alveolar and capillary surface areas available for diffusion, as well as their alveolar barrier’s thickness as an impediment to that diffusion. The subject’s measured value for DLCO then can be compared with expected normative values like other spirometric data to assess the severity of emphysema, edema, interstitial fibrosis, or other disease processes that impact SA, SC, or τS.
It should be emphasized that despite crossing the alveolar-capillary membrane, O2
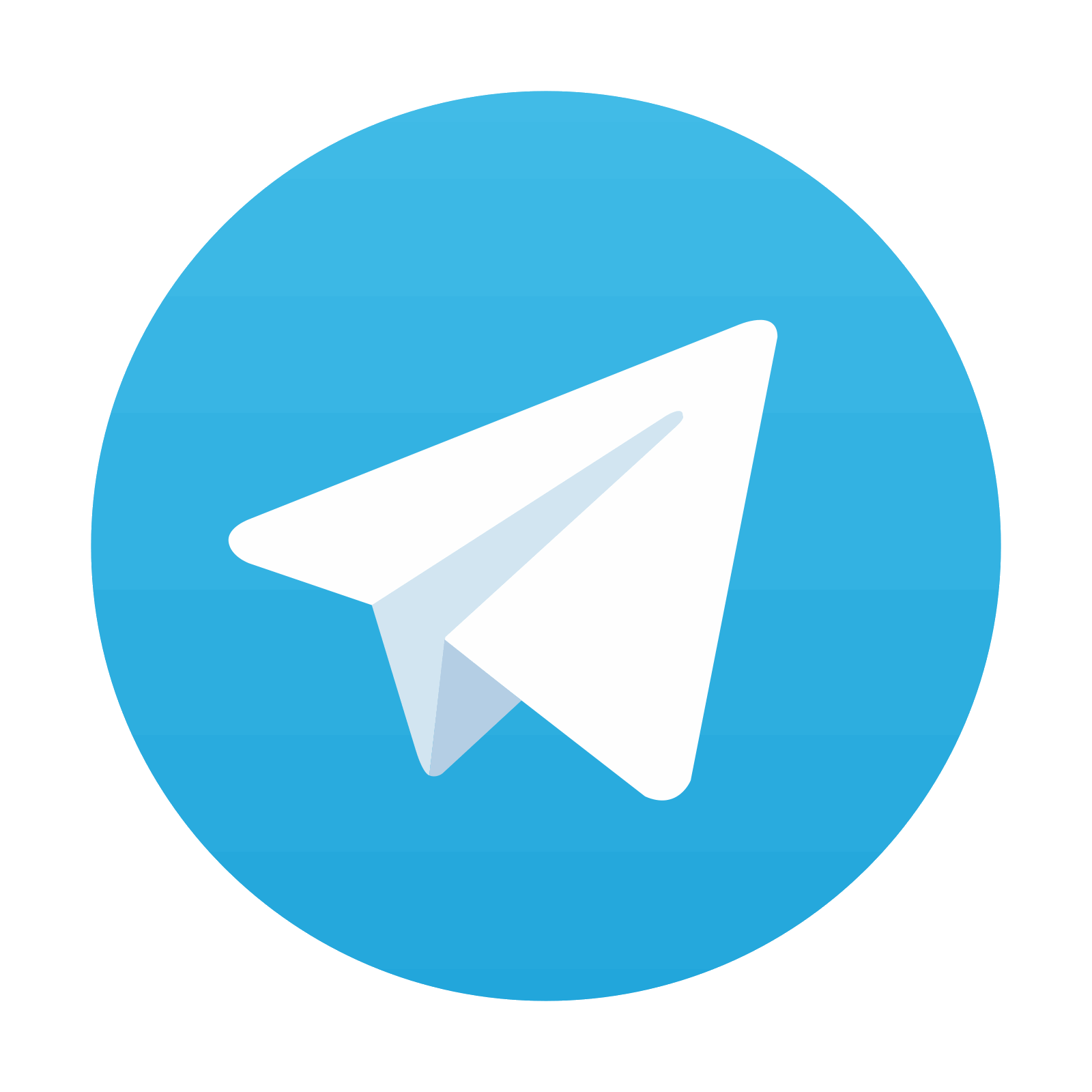
Stay updated, free articles. Join our Telegram channel
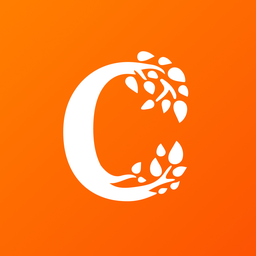
Full access? Get Clinical Tree
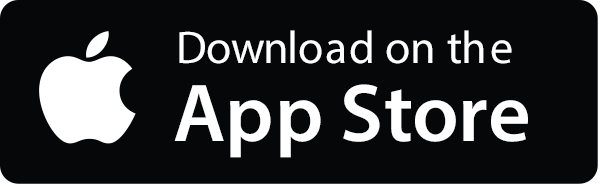
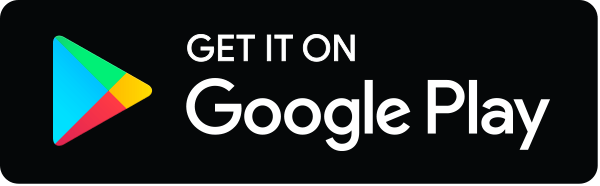
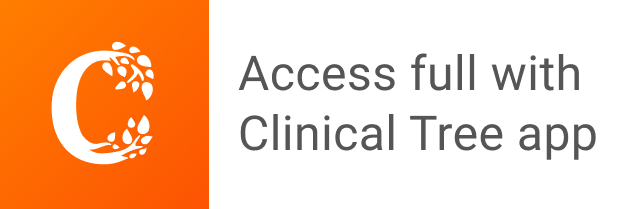