Outline
Left Ventricular Remodeling, 166
Alterations in the Biology of the Cardiac Myocyte, 166
Alterations in the Myocardium, 169
Changes in Left Ventricular Geometry, 170
Clinical Studies Linking Left Ventricular Remodeling With Untoward Patient Outcomes, 170
Reverse Left Ventricular Remodeling, 171
Reversal of Alterations in the Biology of the Cardiac Myocyte, 172
Reversal of Alterations in the Myocardium, 174
Reversal of Changes in Left Ventricular Geometry, 176
Myocardial Remission and Myocardial Recovery, 177
Summary and Future Directions, 180
One of the major conceptual advances in the field of heart failure (HF) has been the recognition that HF progresses because of the structural changes that occur in the heart in response to hemodynamic, neurohormonal, epigenetic and genetic factors. Although the complex changes that occur in the heart during left ventricular (LV) remodeling have traditionally been described in anatomic terms, the process of LV remodeling arises secondary to changes in biology of the cardiac myocyte, histological changes resulting from changes in the volume of myocyte and nonmyocyte components of the myocardium, as well as macroscopic changes in the geometry and architecture of the LV chamber ( Table 12.1 ). Clinical studies have shown that resolution of the cardiac injury that led to myocardial dysfunction and/or the implementation of medical and device therapies that reduce HF morbidity and mortality, results in a reversal of LV remodeling, which is characterized anatomically by a return in LV volume and mass toward normal values, as well as a shift in the LV end-diastolic pressure-volume relationship (EDPVR) to the left. These salutary changes represent the summation of a series of integrated biologic changes in cardiac myocyte size and function, as well as modifications in LV structure and organization. For want of better terminology, these changes have been referred to collectively as reverse remodeling . A small subset of patients experience sufficient reverse remodeling to regain a normal ejection fraction (EF) in a process that has been referred to as myocardial recovery. The rate of normalization in left ventricular ejection fraction (LVEF) depends on the underlying etiology of the cardiomyopathy. Moreover, there is growing body of evidence that suggests that, even among patients who experience a complete normalization in LVEF, there is tremendous heterogeneity and that a significant proportion will have recurrent LV dysfunction and recurrent HF events. The importance of understanding the biology of LV remodeling, reverse LV remodeling, and the subtleties of myocardial recovery is that it may lead to the identification of novel therapeutic targets for treating/reversing HF. In the chapter that follows, we will discuss the changes that occur during the process of LV dilation in HF with a reduced EF. The remodeling that occurs in HF with a preserved EF is discussed in Chapter 11 .
Alterations in Myocyte Biology |
|
Myocardial Changes |
|
Alterations in Left Ventricular Chamber Geometry |
|
Left Ventricular Remodeling
The term left ventricular (LV) remodeling describes the changes in mass, volume, shape, and composition observed in the LV in response to mechanical stimulation and systemic neurohormonal activation. Conceptually, these changes can be envisioned as occurring within the cardiac myocyte, in the histological structure of the myocardium, and within the LV chamber.
Alterations in the Biology of the Cardiac Myocyte
The changes that occur in the biology of the failing cardiac myocyte include (1) cell hypertrophy ( see Chapter 2 ); (2) reactivation of fetal genes; (3) β-adrenergic desensitization ( see Chapter 6 ); (4) loss of myofibrils and progressive disarray of the cytoskeleton; (5) changes in excitation-contraction coupling leading to alterations in the contractile properties of the myocyte ( see Chapter 2 ); and (6) modifications of myocyte metabolism ( see Chapter 17 ). Collectively these changes lead to decreased shortening and delayed relaxation of the failing cardiac myocyte.
Two basic patterns of cardiac hypertrophy occur in response to hemodynamic overload ( Fig. 12.1 ). In pressure overload hypertrophy (e.g., with aortic stenosis or hypertension), the increase in systolic wall leads to the addition of sarcomeres in parallel, an increase in myocyte cross-sectional area, and increased LV wall thickening. This pattern of remodeling has been referred to as concentric hypertrophy (see Fig. 12.1A ) and has been linked with alterations in calcium/calmodulin-dependent protein kinase II-dependent signaling. In contrast, in volume overload hypertrophy (e.g., with aortic and mitral regurgitation), increased diastolic wall stress leads to an increase in myocyte length with the addition of sarcomeres in series, thereby engendering increased LV dilation. This pattern of remodeling has been referred to as eccentric hypertrophy (so named because of the position of the heart in the chest) or a dilated phenotype (see Fig. 12.1A ) and has been linked with Akt activation. Patients with HF classically present with a dilated LV with or without LV thinning. The myocytes from these failing ventricles have an elongated appearance that is characteristic of myocytes obtained from hearts subjected to chronic volume overload. Cardiac myocyte hypertrophy leads to reactivation of portfolios of genes that are normally not expressed postnatally. The reactivation of these fetal genes, the so-called fetal gene program, is also accompanied by decreased expression of a number of genes that are normally expressed in the adult heart including the gene for the β1 adrenergic receptor with resulting β-adrenergic desensitization ( see Chapter 6 ). As will be discussed below, activation of the fetal gene program may contribute to the contractile dysfunction that develops in the failing myocyte in many other ways. The stimuli for the genetic reprogramming of the myocyte include mechanical stretch/strain of the myocyte, neurohormones (e.g., NE, angiotensin II), inflammatory cytokines (e.g., tumor necrosis factor [TNF], interleukin-6 [IL-6]), other peptides and growth factors (e.g., ET), and reactive oxygen species (e.g., superoxide, NO). These stimuli occur both locally within the myocardium, where they exert autocrine/paracrine effects, as well as systemically where they exert endocrine effects.

The early stage of cardiac myocyte hypertrophy is characterized morphologically by increases in the number of myofibrils and mitochondria, as well as enlargement of mitochondria and nuclei ( Fig. 12.2 ). At this stage the cardiac myocytes are larger than normal and have preserved cellular organization. As hypertrophy continues, there is an increase in the number of mitochondria, as well as the addition of new contractile elements in localized areas of the cell. Cells subjected to long-standing hypertrophy show more obvious disruptions in cellular organization, such as markedly enlarged nuclei with highly lobulated membranes, accompanied by the displacement of adjacent myofibrils with loss of the normal registration of the Z bands. The late stage of hypertrophy is characterized by loss of contractile elements (myocytolysis) with marked disruption of Z bands, severe disruption of the normal parallel arrangement of the sarcomeres, accompanied by dilation and increased tortuosity of T tubules.

Failing human cardiac myocytes also undergo a number of other important changes expected to lead to a progressive loss of contractile function, including decreased α-myosin heavy chain gene expression with a concomitant increase in β-myosin heavy chain expression. The cytoskeleton of the myocyte consists of actin, the intermediate filament desmin, the sarcomeric protein titin, and α- and β-tubulin that form microtubules by polymerization. Vinculin, talin, dystrophin, and spectrin represent a separate group of membrane-associated proteins. The failing myocyte shows alterations in cytoskeletal proteins, and in numerous experimental models cytoskeletal and/or membrane-associated proteins have been implicated in the pathogenesis of HF. This is accompanied by alterations in excitation-contraction coupling that have been closely linked to myocardial contractile dysfunction ( see Chapter 2 ). These changes include modification in the abundance of critical Ca 2+ regulatory proteins including sarcoplasmic endoreticular Ca 2+ ATPase (SERCA), ryanodine receptor (RyR), L-type calcium channel (LTCC), and sarcolemmal Na + /Ca 2+ exchanger (NCX). Furthermore, several lines of evidence suggest that the failing myocyte experiences metabolic changes, which leads to impaired efficiency of myocardial energetics ( see Chapter 17 ). When the contractile performance of isolated failing human myocytes was examined under very simple experimental conditions, investigators found that there is an approximately 50% decrease in cell shortening in failing human cardiac myocytes when compared with nonfailing human myocytes.
Alterations in the Myocardium
The alterations that occur in failing myocardium may be categorized broadly into those that occur within the cardiac myocyte compartment, those that occur in the volume and composition of the extracellular matrix (ECM), as well as changes in the myocardial microvasculature. With respect to the changes that occur in the cardiac myocyte component of the myocardium, there is increasing evidence to suggest that progressive myocyte loss may contribute to the development of LV dysfunction and LV remodeling. Necrotic and apoptotic cell death are discussed in Chapter 2 . Whereas the distinction between necrosis and apoptosis is obvious in certain circumstances, the dividing line between these two conditions is often less clear in the failing heart. And indeed, similar mechanisms can operate in both types of cell death. Thus instead of the existence of distinct types of cell death in HF, there is likely a continuum of cell death responses that contribute to progressive myocyte loss and disease progression.
Changes within the ECM constitute the second important myocardial adaptation that occurs during cardiac remodeling and include changes in overall collagen content, changes in the relative contents of different collagen subtypes, changes in collagen cross-linking, and modifications of the connections between cells and the ECM via integrins. Studies in failing human myocardium have shown that there is a quantitative increase in collagen I, III, VI, and IV; fibronectin; laminin; and vimentin, and that the ratio of type I collagen to type III collagen is decreased in patients with ischemic cardiomyopathy. Moreover, clinical studies suggest that there is a progressive loss of cross-linking of collagen in the failing heart, as well as loss of connectivity of the collagen network with individual myocytes, which would be expected to result in profound alterations in LV structure and function. Further, loss of cross-linking of the fibrillar collagen has been associated with progressive LV dilation following myocardial injury. The accumulation of collagen can occur on a “reactive” basis around intramural coronary arteries and arterioles (perivascular fibrosis) or in the interstitial space (interstitial fibrosis), and does not require myocyte cell death ( Fig. 12.3 ). Alternatively, collagen accumulation can occur as a result of microscopic scarring (replacement fibrosis) that develops in response to cardiac myocyte cell necrosis. This scarring or “replacement fibrosis” is an adaptation to the loss of parenchyma and is therefore critical to preserve the structural integrity of the heart. The increased fibrous tissue would be expected to lead to increased myocardial stiffness, which would presumably result in decreased myocardial shortening for a given degree of afterload. In addition, myocardial fibrosis may provide the structural substrate for atrial and ventricular arrhythmias, thus potentially contributing to sudden death. Although the full complement of molecules responsible for fibroblast activation is not known, many of the classical neurohormones (e.g., angiotensin II, aldosterone) and cytokines (ET, transforming growth factor-β [TGF-β], cardiotrophin-1) that are expressed in HF are sufficient to provoke fibroblast activation. And indeed, the use of angiotensin-converting enzyme (ACE) inhibitors, β-blockers, and aldosterone receptor antagonists has been associated with a decrease in myocardial fibrosis in experimental HF models.

Although the fibrillar collagen matrix was initially considered to form a relatively static complex, it is now recognized that these structural proteins can undergo rapid turnover. As discussed in Chapter 4 , one of the more exciting developments with respect to understanding the pathogenesis of cardiac remodeling has been the discovery that a family of collagenolytic enzymes, collectively referred to as matrix metalloproteinases (MMPs), are activated within the failing myocardium. Conceptually, disruption of the ECM would be expected to lead to LV dilation and wall thinning as a result of mural realignment (slippage) of myocyte bundles and/or individual myocytes within the LV wall, as well as LV dysfunction as a result of dyssynchronous contraction of the LV. Although the precise biochemical triggers that are responsible for activation of MMPs are not known, it bears emphasis that TNF, as well as other cytokines and peptide growth factors that are expressed within the failing myocardium, is capable of activating MMPs. However, the biology of matrix remodeling in HF is likely to be much more complex than the simple presence or absence of MMP activation. In fact, degradation of the matrix is also controlled by glycoproteins termed tissue inhibitors of matrix metalloproteinases (TIMPs), which are capable of regulating the activation of MMPs by binding to and preventing these enzymes from degrading the collagen matrix of the heart. The TIMP family presently consists of four distinct members, known as TIMP-1, -2, -3, and -4, each of which is constitutively expressed in the heart by fibroblasts, as well as myocytes. TIMPs-1, -2, -3, and -4 are secreted proteins that act as the natural inhibitors of active forms of all MMPs, although the efficiency of MMP inhibition varies among the different members. The existing literature suggests that MMP activation can lead to progressive LV dilation, whereas TIMP expression favors progressive myocardial fibrosis ( see Chapter 4 ).
The histologic modifications of the failing myocardium are not limited to the ECM but also involve significant changes in the relationship between cardiac myocytes and their blood supply. In fact, although cardiac growth and angiogenesis are tightly coordinated during development and physiologic cardiac growth, following hemodynamic overload and/or cardiac injury it is easy to observe a mismatch between cardiac myocyte growth and blood supply that may lead to contractile dysfunction and cell death. This has been especially well documented in patients with dilated cardiomyopathy that have a reduced myocardial capillary density. Thus impaired capillary growth may contribute to the development and/or progression of HF.
Changes in Left Ventricular Geometry
The changes that occur in the biology of the cardiac myocyte and the myocardium (cardiac myocytes and ECM) lead to progressive LV dilation and increased sphericity of the ventricle. The increase in LV end-diastolic volume, along with LV wall thinning that can occur in some settings, sets the stage for progressive functional ventricular-afterload mismatch that contributes further to a decrease in stroke volume. Moreover, the high end-diastolic wall stress might be expected to lead to (1) hypoperfusion of the subendocardium, with resultant ischemia and worsening of LV function; (2) increased oxidative stress with resultant activation of families of genes that are sensitive to free radical generation (e.g., TNF and interleukin-1β); and (3) sustained expression of stretch-activated genes (angiotensin II, endothelin, and TNF) and/or stretch activation of hypertrophic signaling pathways. Increasing LV dilatation and sphericity also result in tethering of the papillary muscles, resulting in incompetence of the mitral valve and the development of “functional mitral regurgitation.” Whereas the amount of functional mitral regurgitation was once thought to be mild, the advent of noninvasive imaging modalities has shown that functional mitral regurgitation is clinically significant. Apart from the more obvious problem of loss of forward blood flow, mitral regurgitation presents yet a second problem to the heart insofar as the mitral regurgitation results in further hemodynamic overloading of the ventricle. Taken together, the mechanical burdens that are engendered by LV remodeling might be expected to lead to decreased forward cardiac output, increased LV dilation (stretch), and increased hemodynamic overloading, any or all of which are sufficient to contribute to disease progression independently of the neurohormonal status of the patient. Moreover, the aforementioned changes in LV structure and function might be expected to make the cardiovascular system less responsive to normal homeostatic control mechanisms, such as increased adrenergic drive. Thus alterations in the remodeled ventricle may foster a self-amplifying situation in which worsening neurohormonal activation occurs in response to the inability of the remodeled LV to respond appropriately to these compensatory mechanisms. Moreover, at some point in time it is predictable that the aggregate end-organ changes that occur within the cardiomyopathic ventricle may progress to the point that no amount of neurohormonal stimulation can maintain cardiovascular homeostasis, at which point HF may progress independent of the neurohormonal statues of the patient.
Clinical Studies Linking Left Ventricular Remodeling With Untoward Patient Outcomes
Natural history studies have shown that progressive LV remodeling is directly related to future deterioration in LV performance and a less favorable clinical course in patients with HF. White and colleagues were among the first to demonstrate that LV volume had greater predictive value for survival postinfarction than did LVEF. These authors measured LV volumes, LVEF, and severity of coronary arterial occlusions and stenosis at 1 to 2 months after a first or recurrent myocardial infarction. Survivors were followed for a mean of 78 months (range 15–165 months). There were 101 cardiac deaths, of which 71 (70%) were sudden (instantaneous or found dead). Multivariate analysis with log rank testing and the Cox proportional hazards model showed that end-systolic volume had greater predictive value for survival than did end-diastolic volume or EF. Interestingly, the severity of coronary occlusions and stenosis showed additional prediction of only borderline significance. Similar findings were reported by St. John Sutton and associates, who studied LV enlargement after myocardial infarction in an echocardiographic sub-study of the Survival and Ventricular Enlargement (SAVE) Trial in which patients were randomized to placebo or captopril after an acute myocardial infarction. These investigators found that, irrespective of treatment assignment, baseline LV systolic area and the percent change in LV area were strong predictors of cardiovascular mortality and adverse cardiovascular events. At 1 year, LV end-diastolic and end-systolic areas were significantly larger in the placebo than in the captopril group. Moreover, approximately 25% of the patients who survived 1 year experienced a major adverse cardiovascular event. Relevant to the present discussion, patients who experienced an adverse cardiovascular outcome had a greater than threefold increase in LV cavity size than did patients with an uncomplicated course. In a prospective study of 36 patients with dilated cardiomyopathy, Douglas and associates used two-dimensional echocardiography to study the functional consequences of changes in LV size and shape. In this study, nonsurvivors died an average of 11 months after initiation of the study, whereas survivors were followed up for an average of 52 months (range 40–76 months). Survivors had a smaller LV end-diastolic short-axis dimension when compared with nonsurvivors. Moreover, the ratio of short- to long-axis end-diastolic dimensions was more spherical in those with poorer survival (ratio 0.76 vs. 0.68, P < .02). More recently, Vasan and colleagues studied the impact of changes in LV size on mortality in subjects that were followed in the Framingham study. They examined the relation of the LV end-diastolic and end-systolic internal dimensions, as measured by M-mode echocardiography, to the risk of HF in subjects who had not sustained a myocardial infarction and who were free of HF at the time of enrollment. Data were analyzed using a sex-stratified proportional-hazards regression to assess the association between baseline LV internal dimensions and the subsequent risk of HF, after adjusting for age, blood pressure, hypertension treatment, body mass index, diabetes, valve disease, and interim myocardial infarction. HF was diagnosed based on Framingham criteria (two major, or one major and two minor criteria). These investigators found that during an 11-year follow-up period, HF developed in approximately 1.6% of the subjects. The risk-factor-adjusted hazard ratio for congestive HF was 1.47 (95% confidence interval, 1.25–1.73) for each increment of 1 standard deviation in LV end-diastolic dimension (height indexed). Similar results were obtained using LV end-systolic dimension (hazard ratio = 1.43; 95% confidence interval, 1.24–1.65). Vasan and colleagues concluded that an increase in LV internal dimension is a risk factor for congestive HF in men and women who have not had a myocardial infarction. Thus, both the increased LV size and LV sphericity are predictive of untoward outcomes in HF patients with ischemic and dilated cardiomyopathy.
Reverse Left Ventricular Remodeling
The term reverse remodeling was first used to describe the leftward shift in the LV end-diastolic pressure-volume curve of the failing heart following hemodynamic unloading with a ventricular assist device ( Fig. 12.4 ) or a myocardial wrap with latissimus dorsi muscle. An important feature of the decrease in LV size with reverse remodeling was that the change in LV geometry persisted even if the inciting therapy was abruptly stopped, suggesting that the change in properties reflected intrinsic biologic changes in the LV chamber as opposed to changes in LV volume that occur simply in response to a decrease in LV filling pressure. Fig. 12.5 shows that reverse LV remodeling has been observed in a wide variety of clinical settings, even when the HF is quite severe, including viral myocarditis, postpartum cardiomyopathy, or after removal of a cytotoxic agent. A recurring observation in all these clinical studies is that reverse remodeling is associated with an improvement in the clinical manifestations and outcomes in HF, raising the interesting possibility that reverse remodeling is linked mechanistically to the observed improved HF outcomes. Remarkably, this observation held true also when studying patients with the same EF. Patients with mid-range EF (40%–50%) who had entered this category after experiencing reverse remodeling and improvement in LVEF were in fact found to have better outcomes than patients who had mid-range EF but had not experienced reverse remodeling. As illustrated in Fig. 12.5 , three major causes of dilated cardiomyopathy are associated with spontaneous recovery of LV function and reverse LV remodeling, including abnormal energetics, inflammation, and toxic insults. The greatest degree of recovery and/or normalization of LV function was observed in cardiomyopathies associated with abnormal energetics, whereas the cardiomyopathies associated with the least degree of recovery and/or normalization of LV function occurred with myocarditis and postpartum cardiomyopathy. There is also extensive clinical trial–based evidence supporting the potential for reverse remodeling in patients with chronic HF who have received device-based and surgical interventions (reviewed in references 24–26). Importantly, the percentage of patients with recovery of LV function is greater in more recent clinical studies than in older case series, suggesting that the implementation of evidence-based therapies for HF has impacted the natural history of the disease. A full discussion of the natural history of the recovery of LV function and reverse LV remodeling is beyond the intended scope of this chapter; they are discussed elsewhere in considerable detail.


Although the precise cellular and molecular mechanisms that are responsible for the return toward normal LV size and shape during reverse remodeling are not completely understood, there is a fairly consistent biologic theme with respect to the parameters that return toward baseline following pharmacologic or device therapy. As shown in Table 12.2 , there are a series of favorable changes in cardiac myocyte biology, the composition of the myocardium, and the chamber properties of the LV following pharmacologic and device therapies that lead to reverse remodeling.
βBlocker | ACE Inhibitor | ARB | Aldosterone Antagonists | LVAD | CRT | |
---|---|---|---|---|---|---|
Myocyte Defects | ||||||
Hypertrophy | Decreased | Decreased | Decreased | Decreased | Decreased | Decreased |
Fetal gene expression | Decreased | Decreased | Decreased | ND | Decreased | Decreased |
Myocytolysis | Decreased | ND | ND | ND | Decreased | ND |
β-Adrenergic desensitization | Decreased | Decreased | Decreased | ND | Decreased | Decreased |
EC coupling | Increased | Increased | Increased | ND | Increased | Increased |
Alteration of cytoskeletal proteins | ND | ND | ND | Increased | Increased | Decreased |
Myocardial Defects | ||||||
Myocyte apoptosis | Decreased | Decreased | Decreased | ND | Decreased | Decreased |
MMP activation | Decreased | Decreased | Decreased | Decreased | Decreased | Decreased |
Fibrosis | Decreased | Decreased | Decreased | Decreased | Increased a | Decreased |
Angiogenesis | Increased | Increased | Increased | Increased | Decreased | Increased |
Alterations in Ventricular Geometry | ||||||
LV Dilation | Decreased | Stabilized | Stabilized | Stabilized | Decreased | Decreased |
Reversal of Alterations in the Biology of the Cardiac Myocyte
Clinical studies from patients undergoing left ventricular assist device (LVAD) implantation, cardiac resynchronization therapy (CRT), or cardiac contractility modulation have consistently shown a decrease in cardiac myocyte hypertrophy. Regression of myocyte hypertrophy is accompanied by reversal of many of the changes in myocyte biology that occur during adverse LV remodeling, including alterations in excitation-contraction coupling, alterations in the cytoskeleton, β-adrenergic desensitization, and activation of the fetal gene program. Collectively, these changes may explain the significant increase in contractility (maximal calcium-saturated force generation) in cardiac myocytes isolated from hearts that have undergone LVAD support, when compared with myocytes isolated before LVAD support.
The extant literature suggests that many of the changes in gene expression associated with adverse remodeling are reversible with pharmacologic therapies that have been shown to improve patient outcomes. Treatment with ACE inhibitors or angiotensin receptor blockers (ARBs) were associated with an increase in α-MHC and a decrease in β-MHC at the transcriptional level in experimental models of HF. In patients with dilated cardiomyopathy, β-blockade with metoprolol (selective β 1 -blocker) or carvedilol (β 1 -, β 2 -, and α 1 -blocker) inhibited expression of atrial natriuretic peptide (ANP) and β-MHC and restored that of SERCA and α-MHC at the mRNA level ( Fig. 12.6 ). Changes in the abnormal gene expression have also been observed with the use of mechanical circulatory assist devices. For example, when gene expression profiling was performed in bridge-to-transplantation patients before and after LVAD support, there was a significant downregulation of β-MHC, α-SKA, and B-type natriuretic peptide (BNP) in patients with nonischemic cardiomyopathy who received LVAD support, whereas no differences were observed in patients with ischemic cardiomyopathy. In a similar study that employed a proprietary gene array platform, there was a global increase in the expression of a variety of sarcomeric genes in the hearts of LVAD-supported ischemic and nonischemic cardiomyopathy patients, whereas β-MHC mRNA expression was downregulated, consistent with the previous reports. In a select cohort of LVAD patients with clinical myocardial recovery leading to device explantation, mechanical unloading resulted in increased protein expression of myosin heavy and light chains, tropomyosin, and troponins I, C, and T. Moreover, chronic hemodynamic unloading with an LVAD significantly reduced the expression of ANP, BNP, and natriuretic peptide receptor-C mRNA in patients with end-stage HF. Sequencing-based transcriptomic profiling of changes in the expression levels of myocardial mRNA, microRNA (miRNA), and long noncoding RNAs (lncRNA) before and after mechanical support with an LVAD support revealed that less than 5% of mRNAs and miRNAs were normalized after LVAD support, whereas approximately 10% on long noncoding RNAs were improved and/or normalized after LVAD support. Further, the signature of the expression of long noncoding RNAs, but not mRNAs nor miRNAs, distinguished ischemic from nonischemic HF, suggesting a potentially important role for long noncoding RNAs in pathogenesis of HF. LV endomyocardial biopsies obtained from HF patients who underwent CRT revealed a significant increase in α-MHC and a nonsignificant decrease in β-MHC mRNA levels using quantitative real-time PCR. Vayderheyden and colleagues have demonstrated that CRT resulted in increased expression of α-MHC and BNP mRNA levels, and reduced the expression of β-MHC mRNA in patients who improved clinically. Changes in fetal gene expression were not seen in “nonresponders,” defined as failure to improve greater than 1 New York Heart Association (NYHA) functional class score and less than 25% relative increase in EF after 4 months of therapy. In a dog model of HF induced by intracoronary microembolization, therapy with a cardiac support device resulted in a return in β-MHC, α-MHC, ANP, and BNP toward values observed in sham-operated animals. Taken together, these data suggest that both drug and device therapies contribute to reversal of the fetal gene program that has been associated with abnormal contractile function of the cardiac myocyte. However, what is not clear from the extant literature is which of the above changes is/are necessary and/or essential for myocardial recovery.
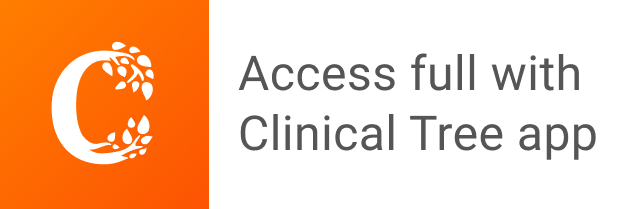