Background
The aim of this study was to use novel area-deformation (ε) loops to interrogate the interaction between the right ventricular (RV) and left ventricular (LV) mechanics following a 100-mile endurance run.
Methods
Fifteen participants (mean body mass, 70.1 ± 8.8 kg; mean age, 40 ± 8 years) were recruited for the study. Echocardiography was performed before the race, after the race, and 6 hours into recovery. RV and LV area and longitudinal ε were assessed using standard and speckle-tracking echocardiography. Following cubic spline interpolation, these variables were obtained across the same cardiac cycle and used to derive area-ε loops.
Results
The RV area-ε loop demonstrated a rightward shift after the race, with increased RV area (from 26.0 to 27.1 cm 2 ) and reduced peak RV ε (from −28.6% to −25.8%). The recovery RV area-ε loop was similar to the postrace loop. A leftward shift was observed in the LV area-ε loop after the race, secondary to reduced LV area (from 35.8 to 32.5 cm 2 ) and reduced peak ε (from −18.3% to −16.6%). In recovery, LV ε values returned toward baseline.
Conclusions
A 100-mile ultramarathon resulted in a rightward shift in the RV area-ε loop as a result of RV dilatation. There was a concomitant leftward shift in the LV area-ε loop as a result of underfilling of the left ventricle. At 6 hours after exercise, there was a partial recovery of the left ventricle, while RV function remained depressed. It appears that changes in RV function do not have a serial impact on the left ventricle during recovery from ultra-endurance activity.
Highlights
- •
This study provides data for temporal cardiac mechanics using area-ε loops.
- •
The loops assess the interaction between the ventricles following prolonged exercise.
- •
There is not a serial impact of the right ventricle on the left ventricle.
- •
This provides further mechanistic understanding of exercise-induced cardiac fatigue.
The impact of prolonged strenuous exercise on cardiac function has received significant attention, with evidence of a transient, negative impact on both the right and left ventricles. A number of theories describing the possible mechanisms responsible for these findings have been proposed, including β-adrenergic receptor desensitization, oxidative stress, and impaired calcium metabolism, but these have yet to be substantiated. A new theory has suggested that right ventricular (RV) function may be depressed after exercise because of the disproportionate changes in RV wall stress, subsequent to increased pulmonary vascular resistance encountered during prolonged activity. In this instance, the right ventricle is unable to maintain contractile force against an elevated afterload, and to sustain stroke volume (SV), the right ventricle dilates. A reduction in RV SV would have the effect of reducing blood volume through the pulmonary system, reducing preload to the left atrium. This in turn would influence overall left ventricular (LV) filling.
Echocardiographic techniques such as strain (ɛ) imaging have allowed a more comprehensive assessment of LV and RV function, and these have recently been used in the post–prolonged exercise setting. These studies have reported reductions in peak LV and RV ε alongside alterations in chamber dimensions, but the impact of ultra-endurance exercise on temporal cardiac mechanics remains largely unknown. In this setting, the interaction of RV and LV structure and function has received limited attention, and a comprehensive evaluation of simultaneous structure and ε throughout the cardiac cycle has not been attempted. The combination of echocardiographic modalities may help reveal mechanical changes in cardiac function while offering a more comprehensive understanding of exercise-related structural and functional adaptation. The concept of assessing area-ε relationships (loops) within the ventricles is novel and provides the potential for determining the contribution of longitudinal deformation to area change in both ventricles.
In view of this, in the present study we used a novel approach by assessing echocardiographically derived temporal area-ε loops in conjunction with conventional two-dimensional (2D) and Doppler indices to establish any serial impact of changes in RV structure and function on LV structure and function as well as any ventricular interaction following prolonged strenuous exercise (a 100-mile endurance run). Furthermore, we aimed to establish whether any changes in cardiac mechanics persist 6 hours into recovery from the exercise bout.
Methods
Sample Population
Fifteen elite runners (14 men, one woman; mean body mass, 70.1 ± 8.8 kg; mean height, 179 ± 6 cm; mean age, 40 ± 8 years) at the 100-mile 2013 Western States Endurance Run (from Squaw Valley to Auburn, CA) were recruited and volunteered to take part in the study. Participants self-reported with no known cardiovascular disease, no prescribed medications, and no comorbidities or family histories of cardiovascular disease. The current training status (mean training days, 6 ± 1 per week; 65 ± 12 miles, 12 ± 3 hours per week) and number of completed ultra-marathons (38 ± 32) were documented. Written informed consent was obtained and ethics approval granted by the Liverpool John Moores University ethics committee.
Protocols
Participants were assessed before the race (24-48 hours before the race) and immediately after the race (within 30 min of race completion). A subsample ( n = 9) also returned for recovery data collection 6 hours after race completion. Height, body mass, resting blood pressure (BP), a resting 12-lead electrocardiogram, and a supine echocardiogram were recorded at each time point. For the prerace assessments, participants were requested to avoid vigorous training, alcohol for a minimum of 24 hours before the initial assessment, and caffeine 4 hours before this time point. Throughout the race, the participants were permitted to consume food and fluid ad libitum, and temperature ranged from 73°F to 102°F. Race finishing time ranged from 18:55 to 23:55 hours.
Echocardiographic Assessments
All echocardiographic images were acquired using a commercially available ultrasound system (Vivid Q; GE Vingmed Ultrasound AS, Horten, Norway) with a 1.5- to 4-MHz phased-array transducer. Images were obtained by a single experienced sonographer with the participant in the left lateral decubitus position. Images were recorded to DVD in raw Digital Imaging and Communications in Medicine format, and data were analyzed offline using commercially available software (EchoPAC version 7; GE Vingmed Ultrasound AS). A minimum of three cardiac cycles were averaged for all peak indices.
Conventional 2D, Doppler, and Tissue Doppler Echocardiography
The right ventricle was assessed in accordance with American Society of Echocardiography guidelines, providing structural and functional indices at the outflow tract (parasternal long axis at the aortic valve level, parasternal short axis at the aortic valve level, and parasternal short axis at the infundibulum) and at the inflow (minor axis at the basal level, minor axis at the midlevel, and major axis). RV diastolic area and systolic area were measured, and the fractional area change was calculated. RV SV was calculated from conventional pulsed-wave Doppler using the volumetric equation, RV SV = (π r 2 ) × (RV outflow tract parasternal short axis at the infundibulum) velocity-time integral, where r = (RV outflow tract parasternal short axis at the infundibulum) (systole) /2, and the velocity-time integral is obtained subvalvular. A pulsed-wave Doppler tissue imaging sample positioned at the tricuspid annulus allowed the assessment of peak myocardial velocities in systole (S′), early diastole (E′), and late diastole (A′). RV systolic pressure was derived from the tricuspid regurgitant jet using continuous-wave Doppler. Pulmonary artery systolic pressure (mm Hg) was calculated as RV systolic pressure + 5 mm Hg. RV and LV end-systolic wall stress was calculated using the formula end-systolic wall stress = Pr /2 h , where P is LV systolic pressure, r is the internal radius, and h is diastolic wall thickness, as previously described.
A comprehensive assessment of LV structure and function was undertaken in accordance with American Society of Echocardiography guidelines. LV end-diastolic volume and end-systolic volume were estimated using the Simpson biplane method, allowing the calculation of SV and ejection fraction. LV eccentricity index was calculated as a measure of interventricular septal displacement. LV diastolic function was assessed using transmitral Doppler providing peak velocities in early (E) and late diastole (A) and their ratio (E/A). Pulsed-wave Doppler tissue imaging assessment of the lateral and septal annulus provided S′, E′, and A′ velocities and the average of both walls reported. The E/E′ ratio was calculated as a noninvasive surrogate of left atrial (LA) pressure.
A full assessment of LA and right atrial structure and volumetric function was performed using the Simpson biplane method, as previously described. LA and right atrial volumes at end-systole, end-diastole, and before the P wave were calculated, allowing the derivation of reservoir, conduit, and booster volumes.
Two-Dimensional Myocardial Speckle-Tracking
A focused apical four-chamber orientation was acquired for assessment of the left ventricle, while a modified image with lateral transducer movement was acquired for assessment of the right ventricle. For the assessment of LV circumferential function, rotation and torsion, images of the left ventricle were acquired from a parasternal short-axis view at the base, mid, and apex. For all images the system was optimized as previously described. Offline analysis allowed the assessment of peak global longitudinal RV ε calculated as an average of three myocardial segments from base to apex of the RV lateral wall. LV global longitudinal ε is based on a six-segment model from the four-chamber view only to allow the construction of simultaneous area-ε loops. Peak global LV circumferential ε was calculated as an average of six myocardial segments at the basal mid and apical levels. Peak basal and apical rotation and rotation rates in systole and early and late diastole were obtained to allow the calculation of peak twist and twist rate as the net difference between basal and apical rotation and rotation rate, respectively.
Area-ε Loops
To standardize for variable heart rates, temporal data were obtained throughout the entire cardiac cycle using cubic spline interpolation in Microsoft Excel 2010 (Microsoft Corporation, Redmond, WA) to provide 300 data points for both systole and diastole, as previously described. The splined data of longitudinal RV and LV ε were used to derive time points for the simultaneous area and ε calculations. Both systole and diastole were divided into 10% increments, essentially providing 20 time points and subsequent ε values across the full cardiac cycle. The original image and cardiac cycle that was used to derive the ε values was then reanalyzed for RV and LV area in two dimensions at each corresponding time point, hence providing simultaneous RV and LV ε, and RV and LV area (see Figure 1 ). This was undertaken for each individual participant, and the mean area-ε at percentage increments was calculated across the cohort. Data were plotted as area against ε (area-ε loop) for the whole cohort for RV and LV longitudinal motion using commercially available software (GraphPad Prism; GraphPad Software, La Jolla, CA).

Polynomial regression analysis of the order y = mx 2 + mx + c was performed on each individual participant’s area-ε loops for systole and diastole independently before the race, after the race, and at 6 hours. Using the polynomial equation, ε values at systole and diastole were calculated for 10% increments of the chamber’s end diastolic area (EDA) within the range 40% to 90% for the left ventricle and 60% to 90% for the right ventricle to reflect physiologic functional area change in each ventricle. The difference between the same percentage of EDA in systole and diastole was calculated and termed the systolic-diastolic ε gradient.
Reliability data for the RV and LV area-ε loops were assessed by a single operator constructing and analyzing individual loops in a separate sample of 20 healthy control subjects on two separate occasions. Data from the right and left ventricles were similar across EDA ranges (40%-80%), with coefficient of variation values ranging from 7% to 21% for simultaneous ε, area, and systolic-diastolic gradient. Comprehensive reliability data for each 10% change in EDA is provided in Supplemental Tables 1-3 .
Statistical Analysis
Because of the reduced sample size from postrace to 6-hour data collection, prerace versus postrace data were compared using Student’s paired t tests, and recovery data are reported for descriptive purposes only. All statistical tests were performed using commercially available software (IBM SPSS version 21; IBM, Armonk, NY). Previous studies on a similar sample size have set α as P < .05 with no correction for multiple comparisons; in the present study, α was set at P < .01 as a sensible balance between the likelihood of producing type I and II statistical errors.
Results
Demographics
Systolic and diastolic BPs were significantly reduced after the race. Heart rate and body mass were not different at the pre- and postrace assessments (see Table 1 ).
Parameter | Before race ( n = 15) | After race ( n = 15) | Recovery ( n = 9) |
---|---|---|---|
Body mass (kg) | 70.1 ± 8.8 | 68.8 ± 7.8 ∗ | 66.1 ± 7.9 |
Systolic BP (mm Hg) | 134 ± 11 | 114 ± 12 ∗ | 117 ± 12 |
Diastolic BP (mm Hg) | 84 ± 10 | 76 ± 8 ∗ | 77 ± 8 |
Heart rate (beats/min) | 63 ± 10 | 70 ± 10 | 71 ± 12 |
∗ Statistical significance between prerace and postrace measures ( P < .01).
Conventional 2D, Doppler, and Tissue Doppler Echocardiography
There was a postrace increase of 13% in RV outflow and inflow dimensions ( P = .004 and P = .002, respectively; see Table 2 ), and there was an 18% reduction in RV S′ ( P = .005; Table 2 ). RV SV was maintained, with no significant reduction observed after the race, as were RV fractional area change and RV E′. RV wall stress was elevated compared with baseline immediately after the race and in recovery, and pulmonary artery pressure was reduced after the race compared with prerace measures, albeit not significantly. There was an 11% decrease in LV end-diastolic volume after the race ( P = .005; see Table 2 ). There was an 18% decrease in transmitral E ( P = .001) and a subsequent 19% decrease in the E/A velocity ratio ( P = .003). LV S′, E′, and A′ were reduced by 10% after the race ( P < .006). LV wall stress was reduced after the race and in recovery compared with prerace values, albeit not significantly. LA volume at end-systole, before the P wave, and at end-diastole and conduit, reservoir, and booster volumes were not different after the race ( P > .01). There was no change in right atrial volume at end-systole, before the P wave, and at end-diastole and reservoir and booster volumes from before to after the race ( P > .01).
Parameter | Before race ( n = 15) | After race ( n = 15) | Recovery ( n = 9) |
---|---|---|---|
Right ventricle | |||
RVOT plax (mm) | 30 ± 4 | 33 ± 3 ∗ | 33 ± 4 |
RVOT 1 (mm) | 32 ± 4 | 36 ± 4 ∗ | 35 ± 5 |
RVOT 2 (mm) | 25 ± 2 | 28 ± 2 | 27 ± 3 |
RVD 1 (mm) | 43 ± 4 | 48 ± 5 ∗ | 47 ± 6 |
RVD 2 (mm) | 32 ± 3 | 37 ± 3 ∗ | 36 ± 3 |
RVD 3 (mm) | 84 ± 6 | 83 ± 7 | 82 ± 6 |
RV FAC (%) | 54.1 ± 5.8 | 48.8 ± 4.7 | 50.3 ± 8.2 |
TAPSE (mm) | 24 ± 4 | 23 ± 4 | 26 ± 3 |
RV S′ (cm/sec) | 17 ± 3 | 14 ± 3 ∗ | 16 ± 1 |
RV E′ (cm/sec) | 17 ± 2 | 14 ± 4 | 14 ± 3 |
RV A′ (cm/sec) | 13 ± 5 | 12 ± 3 | 13 ± 3 |
RV SV (mL) | 92 ± 25 | 89 ± 25 | 102 ± 35 |
PASP (mm Hg) | 25 ± 4 | 22 ± 8 | 23 ± 2 |
RV wall stress (kdyne/cm 2 ) | 3.97 ± 1.93 | 4.39 ± 1.30 | 2.94 ± 2.24 |
Left ventricle | |||
LV EDV (mL) | 123 ± 15 | 109 ± 16 ∗ | 112 ± 17 |
LV ESV (mL) | 41 ± 5 | 47 ± 9 ∗ | 39 ± 8 |
LV SV (mL) | 82 ± 11 | 63 ± 11 ∗ | 73 ± 11 |
LVEF (%) | 66 ± 3 | 58 ± 6 ∗ | 65 ± 3 |
MV E (m/sec) | 0.84 ± 0.17 | 0.69 ± 0.18 ∗ | 0.74 ± 0.17 |
MV A (m/sec) | 0.50 ± 0.09 | 0.51 ± 0.11 | 0.53 ± 0.08 |
MV E/A ratio | 1.70 ± 0.38 | 1.37 ± 0.37 ∗ | 1.38 ± 0.26 |
LV S′ (cm/sec) | 13 ± 2 | 12 ± 1 ∗ | 13 ± 2 |
LV E′ (cm/sec) | 16 ± 2 | 13 ± 3 ∗ | 15 ± 2 |
LV A′ (cm/sec) | 10 ± 1 | 9 ± 2 ∗ | 9 ± 2 |
E/E′ ratio | 5.29 ± 1.01 | 5.20 ± 1.05 | 5.11 ± 1.26 |
EI in diastole | 1.16 ± 0.11 | 1.22 ± 0.10 | 1.14 ± 0.08 |
EI in systole | 1.09 ± 0.07 | 1.15 ± 0.12 | 1.14 ± 0.08 |
LV wall stress (kdyne/cm 2 ) | 16.82 ± 2.34 | 14.42 ± 2.40 | 12.83 ± 1.66 |
Left atrium | |||
LA VOL ES (mL) | 55 ± 8 | 57 ± 11 | 60 ± 12 |
LA VOL pre A (mL) | 33 ± 5 | 34 ± 8 | 37 ± 10 |
LA VOL ED (mL) | 17 ± 3 | 21 ± 5 | 22 ± 6 |
LA RES (mL) | 38 ± 6 | 36 ± 6 | 38 ± 8 |
LA CON (mL) | 44 ± 13 | 26 ± 8 | 35 ± 5 |
LA BOO (mL) | 16 ± 74 | 13 ± 4 | 15 ± 6 |
Right atrium | |||
RA VOL ES (mL) | 62 ± 23 | 62 ± 14 | 58 ± 22 |
RA VOL pre A (mL) | 42 ± 14 | 46 ± 13 | 40 ± 13 |
RA VOL ED (mL) | 28 ± 9 | 29 ± 11 | 27 ± 8 |
RA RES (mL) | 34 ± 15 | 33 ± 9 | 31 ± 15 |
RA BOO (mL) | 14 ± 7 | 17 ± 8 | 14 ± 7 |
∗ Statistical significance between prerace and postrace measures ( P < .01).
Myocardial ε Imaging
Peak RV longitudinal ε was reduced by 10% from before to after the race ( P = .007). LV longitudinal ε was reduced by 9% after the race ( P = .01). LV basal, mid, and apical circumferential ε were all reduced (by 19%, 14%, and 15%; P = .001, P = .008, and P = .01, respectively) from before to after the race, as were basal and apical rotation, twist, and systolic and diastolic twist rates (by 39%, 46%, and 46%; P = .007, P = .002, P < .001, P = .004, and P < .001, respectively; see Table 3 ).
Parameter | Before race ( n = 15) | After race ( n = 15) | Recovery ( n = 9) |
---|---|---|---|
RV longitudinal ε (%) | −28.6 ± 3.8 | −25.8 ± 2.8 ∗ | −27.4 ± 4.1 |
LV longitudinal ε (%) | −18.3 ± 1.5 | −16.6 ± 2.7 ∗ | −18.5 ± 2.4 |
LV basal circumferential ε (%) | −22.7 ± 2.0 | −18.5 ± 3.7 ∗ | −21.2 ± 2.4 |
LV mid circumferential ε (%) | −20.4 ± 3.3 | −17.6 ± 3.6 ∗ | −21.1 ± 2.7 |
LV apical circumferential ε (%) | −39.1 ± 8.1 | −33.2 ± 6.6 ∗ | −35.9 ± 7.3 |
Basal rotation (deg) | −8.7 ± 3.5 | −5.3 ± 3.1 ∗ | −5.2 ± 3.2 |
Apical rotation (deg) | 16.5 ± 6.0 | 9.0 ± 5.0 ∗ | 11.7 ± 3.2 |
Twist (deg) | 24.8 ± 6.6 | 13.5 ± 6.3 ∗ | 16.5 ± 3.7 |
Systolic twist rate (deg/sec) | 121.7 ± 25.9 | 90.1 ± 25.8 ∗ | 120.3 ± 16.3 |
Early diastolic twist rate (deg/sec) | −150.6 ± 26.1 | −83.8 ± 33.6 ∗ | −149.3 ± 38.5 |
Late diastolic twist rate (deg/sec) | −79.0 ± 20.9 | −78.5 ± 41.3 | −81.9 ± 33.1 |
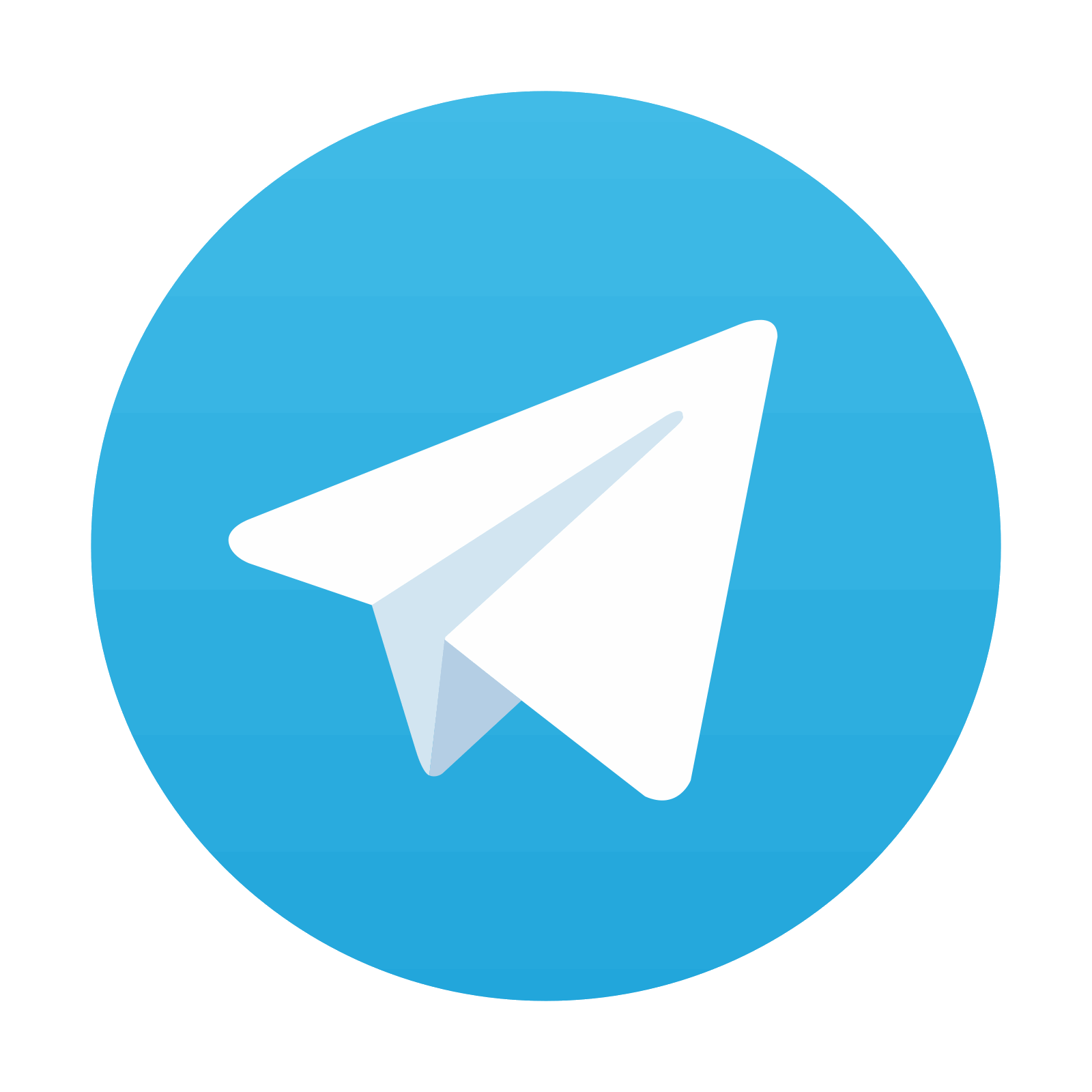
Stay updated, free articles. Join our Telegram channel
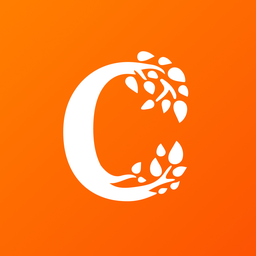
Full access? Get Clinical Tree
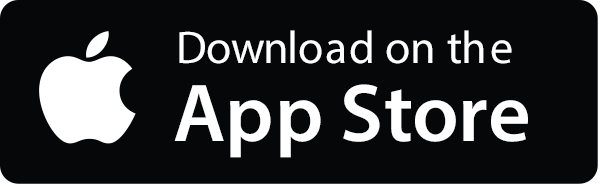
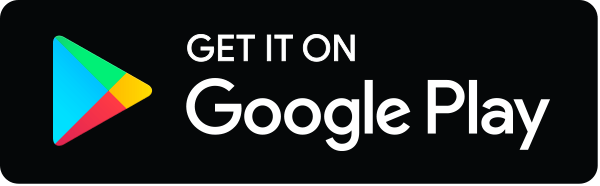
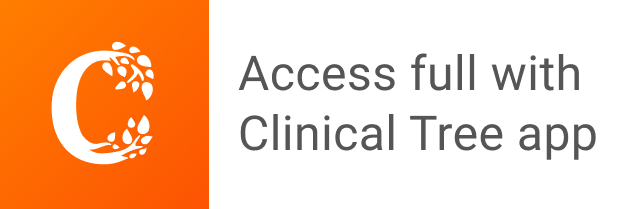