(1)
Project-team INRIA-UPMC-CNRS REO Laboratoire Jacques-Louis Lions, CNRS UMR 7598, Université Pierre et Marie Curie, Place Jussieu 4, 75252 Paris Cedex 05, France
Abstract
Mucosa is a lining involved in absorption and secretion of mucus, especially, among other materials. Mucosal surface comprises a wet epithelium, a structural barrier, covered by a protective mucous barrier. The mucus layer in airways is thin and mobile.
Mucosa is a lining involved in absorption and secretion of mucus, especially, among other materials. Mucosal surface comprises a wet epithelium, a structural barrier, covered by a protective mucous barrier. The mucus layer in airways is thin and mobile.
Human conducting airways are mostly lined with a pseudostratified, secretory, and ciliatedepithelium that comprises 3 major cell types — ciliated, secretory, and basal cells — with submucosal glands and cartilaginous elements in large bronchi (Vol. 1 – Chap. 3. Cells of the Ventilatory Tract).
The lung resists to damages caused by the body’s outside environment following a permanent exposure to pathogens and more or less toxic chemical pollutants in inhaled air. Airway mucus represents the first line of defense. Inhaled particles (allergens, dust, microorganisms, and cellular debris) trapped in the mucus gel are removed from the respiratory tract by the mucociliary clearance. In fact, airway mucus traps inhaled toxins and transfers them out of the airways not only using mucus transport that results from ciliary beats, but also cough. Mucus cleans airways, as it flows from either the tracheobronchial tree or upper airways toward the pharynx, where it is swallowed (or expectorated).
The airway surface liquid consists of 2 components: (1) a gel-like mucus layer that traps inhaled particles and transports them out of airways by a cilia-generated motion and (2) a periciliary liquid layer that supports ciliary beating, hydration of airway mucus, and lubrication of the wetted epithelial surface. Cilia of respiratory epithelia beat in a coordinated manner within a periciliary fluid underneath a layer of mucus gel and transiently impact the mucus layer during the beating cycle, thereby avoiding an oscillatory motion. The luminal gel comprises water and heavily glycosylated mucins.
Pulmonary epithelial cells provide a mechanical barrier to microbial entry and participate in innate defense. They sense the presence of microbes and then augment their barrier function to resist to microbial penetration, signal to leukocytes, release of antimicrobial peptides, and kill pathogens [1511].1 In the nearly sterile distal respiratory tract of healthy lungs, innate immune epithelial function is reduced due to small constitutive stimulation, when the mucociliary clearance functions effectively.
Secretory cells include Clara, goblet, and serous cells (Sect. 12.3.1). Various endo- and exogenous factors modify the secretory cell phenotype. Therefore, the overall term “secretory cell” is more appropriate to designate non-ciliated cells that liberate materials in lumens of the respiratory tract. Secretory cells release mucins as well as antimicrobial (defensins, lysozyme, and immunoglobulin-A), immunomodulatory (secretoglobins and cytokines), and protective (stable, secretory trefoil proteins and heregulin) molecules, either constitutively and inducibly, that can be incorporated into mucus [1511]. In large airways (internal caliber > 2 mm), submucosal glands located betweenairway smooth muscle and cartilage and connected to the airway lumen by a duct (ciliated in its downstram segment) contribute to the secretion of mucins and liquid.
Mucus is a viscoelastic fluid (thickness 2–5 μm) secreted by the respiratory epithelium. It not only traps inhaled particles (allergens, carcinogens, dust, microorganisms, and inflammatory debris) that come into contact with it to clear them from airways, but also protects the mucosa of thetracheobronchial tree from dehydration.
Ciliary motions that are associated with mucus propulsion in the human respiratory tract were described in the nineteenth century by Purkinje and Valentin (1834) and Sharpey (1835). The human respiratory tract and its related cavities and conduits (nose, sinuses, Eustachian tubes, middle ear, pharynx, trachea, and bronchi down to terminal bronchioles) have mucosal surfaces that exhibit mucus flow toward the esophagus. Mucus is more or less continuously secreted, shed, and recycled, discarded, or degraded.
Mucus delivery and motion are investigated in the framework of flow and transport of gases and inhaled particles in the respiratory tract under several alternative modes of ventilation together with aerosol transfer and surfactant dynamics, especially in the terminal airways, where it yields alveolar stability and increased lung compliance.
12.1 Double-Layered Airway Surface Fluid
The liquid layer at the luminal surface of the respiratory epithelium has 2 strata: (1) a deep, more aqueous, glycoprotein-free, lubricating region that bathes cilia (interciliary space ∼ 200 nm) — the epithelial lining fluid — that is also called the periciliary liquid (thickness ∼ 7 μm) and (2) a superficial, viscous domain, the gel or mucus layer.
Membrane-bound mucins contribute to the physical properties of liquid near the cell wetted surface. They confer the features of an anchored gel rather than a simple gel on top of a liquid. Mucin-4 is densely expressed on cilia and yields a brush-like configuration. Tethered mucins form an endoluminal brush [1512]. This brush layer establishes a mesh that prevents mucins of the mucus gel layer as well as deposited inhaled particles to penetrate into the periciliary space and causes mucus to form a distinct layer. Moreover, it provides lubrication through bound water.
In the current gel-on-liquid mucus clearance model, a mucus gel is propelled on top of a periciliary fluid layer over the respiratory epithelium that hence baths cilia. In the gel-on-brush model, the periciliary layer is occupied by membrane-spanning mucins and mucopolysaccharides tethered to the airway wall wetted surface (cilia, microvilli, and epithelial surface; mesh structure 20–40 nm) [1513]. The relatively high concentration of membrane-tethered mucins in the endoluminal brush layer produces intermolecular repulsion within this layer, which stabilizes the periciliary liquid against compression by an osmotically active mucus layer. The relative osmotic moduli of the mucus ( ∼ 200 Pa) and periciliary brush ( > 300 Pa) layers explain that the mucus layer acts as a reservoir for water in the periciliary liquid, thereby stabilizing the mucus clearance in healthy airways [1513]. In airway diseases, when the airway surface is sufficiently dehydrated (the partial osmotic modulus of the mucus layer exceeds the minimal modulus of the periciliary brush), the mucus layer compresses the periciliary brush and cilia, slowing down and eventually stopping mucus clearance [1513]. Immobile mucus favors chronic inflammation and bacterial infections. Increase in the partial osmotic modulus of the mucus layer results from either a decrease in the amount of water solvent, as in mucovicidosis, or an increase in content of secreted mucins as in chronic obstructive pulmonary diseases.
The mucus layer is needed for particle transport. Cilia beat depends mostly on stimulation. The epithelial lining fluid is more stable than the overlying mucus layer, the latter being replaced every 10 to 20 mn. Each layer has a corresponding glandular apparatus: mucoid and serous secretions for the superficial and deep layer, respectively. Cilia freely beat in deep sol layer, but their tips contact the overlying gel layer. The mucus layer entraps all deposited particles that are transported by coordinated ciliary movements. The superficial gel layer and deep fluid layer are separated bysurfactant.
Furthermore, airway surface liquid can be decomposed into 3 compartments when the glycocalyx coating of the apical cell surface is added to the epithelial lining fluid and the overlying mucus layer. Carbohydrate-rich glycocalyx contains intrinsic glycolipids, glycoproteins, and proteoglycans as well as adsorbed glycoproteins and proteoglycans.
The height of the airway surface liquid layer varies from about 3 μm over goblet or non-ciliated brush cells to 7 μm over ciliated cells (layer height is only estimated due to measurement disturbance) [1514].
The lubricating layer contains tethered mucins and other molecules such as cell surface glycolipids, glycoproteins, and proteoglycans, whereas the mucus layer consists of high-molecular-weight mucin dimers and trimers (length 0.5–20 μm) that interact with crosslinkers and innate defense proteins.
12.1.1 Control of the Quantity and Composition of the Airway Surface Fluid
Mechanism of mucus transport thus depends on interaction between the near-wall environment and overlying mucus, regulation of mucus hydration, and mucus adhesion. Electrolyte transport through and between airway epithelial cells controls the quantity and composition of the airway surface liquid.
Water transport across the apical plasma membrane of respiratory epithelial cells, i.e., the hydration status of airway surface liquid, indeed relies on ion fluxes, mainly chloride ion export through both cysticfibrosis transmembrane conductance regulator (CFTR) andcalcium-activated chloride channels (CaCC) as well as sodium import throughepithelial Na + channel (ENaC; Fig. 12.1).
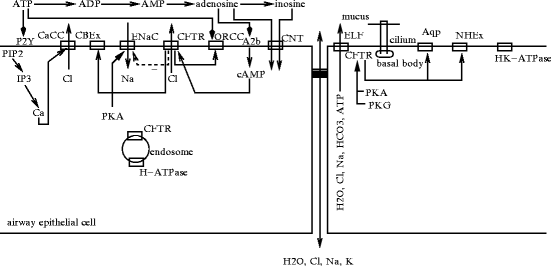
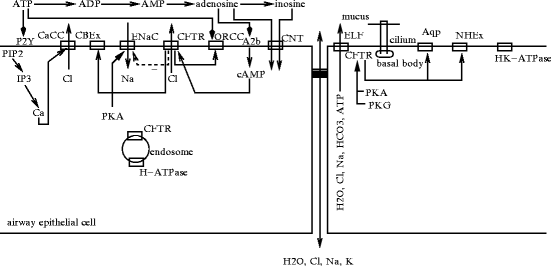
Fig. 12.1
Ion transport across the apical plasma membrane of airway epithelial cells (Source: [1514]). The liquid layer at the luminal surface (airway surface liquid) of the respiratory epithelium has 2 strata: epithelial lining fluid (ELF) and mucus layer. The respiratory cilium beats in epithelial lining fluid, but its tip contact overlying mucus. Aquaporins and ion channels that regulate water and ion transport are involved in production and maintenance of epithelial lining fluid as well as mucus homeostasis. Chloride ions exit through cystic fibrosis transmembrane conductance regulator (CFTR) are stimulated by adenosine binding to its receptor A2B or calcium ions that excite Ca2 + -activated Cl − channels (CaCC) after Ca2 + influx upon stimulation of nucleotide receptor P2Y2 by ATP messenger. Channel CFTR also transports transport ATP, sodium, bicarbonate ions, and water. Channel CFTR is activated by protein kinase-A (PKA), cAMP, and ATP binding. In addition, CFTR is phosphorylated by protein kinases PKG1a and PKG2. Sodium ions are imported through epithelial Na + channel (ENaC). Chloride channel CFTR inhibits ENaC channel. It also regulates Cl − –HCO3 − exchanger (CBEx) and outwardly rectifying Cl − channels (ORCC). Airway surface liquid pH is regulated by H + –K + ATPase and Cl − –HCO3 − exchanger. Channel CFTR can activate aquaporin (Aqp). It also targets regulator NHERF of Na + –H + exchanger NHE3 (NHEx). Channel CFTR located in membrane of endosomal vesicles can counterbalance the activity of H + ATPase. Adenosine and inosine are removed from airway surface by concentrative nucleoside transporters (CNT).
Water flux across the wetted surface of the respiratory epithelium is controlled not only by motions of Cl − across the apical membrane, but also K + flux across the basolateral membrane. Both Cl − and K + conductances of airway epithelial cells are influenced by calcium ions. Conversely, features of the extracellular fluid play a role in calcium signaling [1515].
Two parallel routes — the trans- and paracellular paths — are used for the transepithelial electrolyte transport that determine the quantity and composition of the airway surface liquid and gel. The transcellular route depends on distinct populations of active and passive ion channels, transporters, and pumps in apical and basolateral membranes. Two main transcellular mechanisms of active ion transport comprise ENaC-dependentNa + absorption and CFTR-dependent anion (Cl − andHCO3 − ) secretion. On the other hand, the passive paracellular ion transfer depends on adhesion plaques between apposed epithelial cells that confer the permeability and ion selectivity of the paracellular path. In polarized respiratory epithelia, the paracellular pathway is limited bytight junctions and scattered adherens junctions. The latter localize in the lateral intercellular spaces, where adjacent epithelial cells interdigitate. Circumferential tight junctions form the functional and structural border that separates apical and basolateral compartments. Like many other epithelia, respiratory epithelia produce multipleclaudins, the primary determinants of paracellular ion conductance.2 Rapid regulation of transcellular ion transport occurs in airway epithelia. Several hormones, neurotransmitters, and other agents regulate ion transport through the cell. On the other hand, paracellular ion transfer relies on dilation and disruption of tight junctions that result from the contraction of the actomyosin ring of the cortical cytoskeleton. Like the transcellular ion transport, the paracellular ion transfer can change quickly [1516].Histamine that can be released by mastocytes binds to its H1 receptor and provokes a Ca2 + influx, hence promoting cell contraction, and a rapid and transient increase in the paracellular Na + conductance, in addition to a smaller elevation in Cl − conductance.
Airway surface liquid hydration is also controlled by extracellular nucleotides, such asadenosine triphosphate,uridine triphosphate, and UDP, and nucleosides such asadenosine. These substances bind to cognate receptors. Nucleotides ATP and UTP target nucleotides (purinergic)P2Y2 receptors; UDP binds to receptor P2Y6; adenosine interacts withA2B receptor to stimulate Cl − export into the airway lumen (Vol. 3 – Chap. 7. G-Protein-Coupled Receptors). Moreover, activated P2Y2 inhibits Na + absorption by airway epithelial cells. Nucleotides receptors thus participate in the regulation of airway surface liquid volume. In normal conditions, the respiratory epithelium adjusts Na + and Cl − fluxes so that stretched cilia are almost completely bathed in the lubricating epithelial lining fluid. In the airway lumen, released ATP is converted into adenosine that binds to receptor A2B (Fig. 12.1).
Cyclic motion of the airway wall that is associated with successive lung inflation and deflation regulates homeostasis of airway surface liquid via nucleotides and nucleosides [1514].Aerodynamical stress applied to the airway wall activates P2Y2 receptors to increase luminal ATP level. Oscillatory motion in vitro that mimics tidal volume breathing in vivo favors airway surface liquid production, at least partly, because of ATP-stimulated nucleotide receptor-mediated ENaC inhibition and CaCC activation.
Airway surface liquid pH influences activity of ion channels, mucus adhesion to membranes, and attachment of bacteria and viruses to mucus. The glycocalyx pH is supposed to be influenced by negative charges of sugar moieties and membrane phospholipid head groups that can attractH + ion. In addition, glycoaminoglycans and glycoproteins are sialiated and sulfated, thereby accumulating cations on their surfaces. In healthy airways, airway surface liquid pH is regulated by H + –K + ATPase and CFTR-mediatedHCO3 − flux throughCl − –HCO3 − exchanger.
12.2 Mucociliary Clearance
Mucociliary clearance is a defense mechanism that prevents accumulation of inhaled contaminants within the respiratory tract. Mucociliary clearance that comprises cough results from continuous flow of airway surface liquid over surfaces of airway lumens. Usually, airway surface liquid is cleared by cilium-mediated mucus transport. Normal daily volume of respiratory secretion that arrives at the larynx is estimated to be about 10 ml, as a part of its water content is reabsorbed via ion transport.
In healthy non-smoking adults,tracheal mucociliary clearance rate can be assessed by a radioisotope technique (70–85 μm/s; i.e., from 4.3 ± 1.1 mm/mn [1518] to 5.1 ± 2.9 [1517]). However, mucociliary clearance rates of 100 to 300 μm/s have been measured in the human trachea, i.e., nearly an order of magnitude greater [1519]. In the latter study, intersubject variations of mucus transport rate is not correlated to variations in ciliary beat frequency of nasal cells of examined subjects (12.1 ± 1.7 Hz) measured in vitro.
12.2.1 Factors of the Mucociliary Clearance
Four main factors determine efficiency of basic mucociliary clearance: (1) number of cilia that synchronously beat; (2) cilium beating amplitude and frequency (i.e., velocity of cilium tips); (3) thickness of serous fluid and mucus layers produced by surface goblet cells and submucosal glands; and (4) composition and rheology of serous fluid and mucus (i.e., ion transport and associated epithelial water flux as well as macromolecule content and its degree of entanglement and density of protein crosslinking).
Efficient mucociliary transport requires appropriate mucus composition for optimal flow, i.e., appropriate epithelial water and ion transport and mucin secretion, adequate numbers of functioning ciliated cells and their coordinated motion for mucus propulsion, suitable airway surface liquid depth, as well as respiratory epithelium integrity over long distances. Efficient transfer of momentum between cilia and mucus requires that cilia contact mucus during forward stroke, but minimally interact with it during return. When epithelial lining fluid is either too deep or too shallow, the mucociliary clearance rate decreases. Moreover, ciliary activity is adjusted in response to varying environmental conditions (Sect. 12.6.2).
Hyaluronan, or hyaluronic acid, a glycosaminoglycan lubricant at the airway epithelium surface, enhances the transport of airway mucus by cilia and by cough as well as protects the airway epithelial barrier; the lower the hyaluronan molecular weight, the stronger the effect [1520]. Low-molecular-weight hyaluronan (40 kDa) increases the expression of tight junction proteins such as ZO1 as well as the gap junction function.
12.2.2 Cough Clearance
Air flow–mucus interaction becomes important in clearing respiratory mucus as a lung disease develops. Moreover, various lung diseases are characterized by mucus hypersecretion and impaired airway clearance. Hence, excess mucus must be eliminated by coughing that propels mucus layer parts.
Efficiency of cough clearance depends on mucus rheology and surface properties at both air–mucus and epithelial lining fluid–mucus interfaces. Strong mucus elasticity that favors recoil after cough impedes mucus propulsion toward the larynx. High adhesivity precludes cough clearance. In addition, mucus sheared by strong expiratory air flow during coughing is prevented from sedimenting, as it rapidly recovers its rheological features and does not undergo tearing.
12.2.3 Mucociliary Clearance and Infection
The mucociliary clearance is a major defense process of the airway epithelium against inhaled pathogens and noxious entrapped micro- and nanoparticles that are removed with the mucus layer from the respiratory tract. As an innate airway defense mechanism, the mucociliary clearance affects drug delivery to airways. Drug absorption depends on duration during which drug is retained, i.e., the rate of mucus transport that is usually slowed down in airway pathologies. Engineered nanoparticles must be able to cross viscoelastic and adhesive mucus barrier, limit interactions with mucus constituents, and avoid adhesion and rapid clearance to be delivered in target cells.
Among normal volunteers exposed to rhinovirus or influenza-B virus, some remain uninfected, whereas others experience subclinical or symptomatic infection. Most symptomatic infected volunteers have prolonged nasal clearance, and less than 50% of their epithelium ciliated, but without significant reduction in ciliary beat frequency [1521].
12.2.4 Alterations in Mucociliary Clearance
Altered mucus composition causes abnormal mucociliary transport. Dysfunctional ion channels, such as CFTR and ENaC, that generate depletion of airway surface liquid produce mucus adhesion that favors formation of mucus plaque and plug, thereby an attenuated clearance of inhaled pathogens. Defective chloride transport across mucous membranes prevents normal hydration of mucus, leading to accumulation of highly viscous mucus such as that observed in cystic fibrosis. Mucus-generated clogging in airways, inflammation, and infection cause structural changes in lungs and sinuses. Mucus with elevated viscosity reduces air flow and fails to adequately clear germs.
Loss of water and salt in the airway surface liquid induces collapse of both the lubricating and mucus layers and mucus adhesion to cell wetted surface. On the other hand, addition of water and electrolytes to the airway surface liquid swells the mucus layer, but maintains apposition of both strata.
Ciliary activity that depends on appropriately hydrated airway surface liquid is a dominant factor that govern mucus clearance. In fact, individuals with mucoviscidosis, hence depleted airway surface liquid, quickly develop severe airway infection, whereas asthma that is associated with mucin hypersecretion is not necessarily associated with infection.
Primary ciliary dyskinesia causes impaired coordination of ciliary beating. Primary ciliary dyskinesia (Kartagener’s, or immotile cilia syndrome) causes impaired coordination of ciliary beating. This disease causes a chronic cough, recurrent infections of the lung (bronchitis and pneumonia), and bronchiectasis, as well as chronic sinusitis and otitis.
Leukocyte migration through mucus during mucosal invasion by pathogens is a component of the mucosal immune response. Neutrophils can rapidly travel through mucus [1522]. In the absence of stimulation, random motility of neutrophils and lymphocytes is similar in collagen gels that have a structure comparable to mucus, whereas monocytes remain immotile. Motility of these cells is primed or enhanced upon suitable excitation. Neutrophils are able to cross a gel layer of thickness less than 500 μm.
Immunoglobulins are small enough to diffuse through the mucus mesh. Nevertheless, IgM diffusion is slowed by mucus, as immunoglobulins fabricate low-affinity bonds with mucins [1523]. Nonetheless, IgA, IgG, and IgM diffuse only slightly less in mucus than in water (0.7 <
(mucus)/
(water) < 1.0;
: diffusion coefficient) [1524].



Small viruses (size 38–55 nm) are able to diffuse through mucus as rapidly as in saline because mesh spacing between mucin fibers is large enough (20–200 nm; estimated hydrodynamic pore size for cervical mucus ∼ 100 nm [1523, 1524]). However, herpes simplex virus (180 nm) can make low-affinity bonds with mucins that slow its running rate.
Mucus composition can be altered during infection. Moreover, after respiratory infections, accumulation of neutrophils and cellular debris raises mucus viscosity and impedes lung clearance and sinus drainage. Impaired mucociliary transport is often associated with viral or bacterial respiratory infections. Viral infections can reduce ciliary beating frequency as well as number of cilia and ciliated cells. Among viruses, influenza viruses destroy ciliated respiratory epithelia. Inhaled toxins from cigarette smoking and pollution depress ciliary function.
Purulent sputum from bronchitic patients characterized by a higher viscosity and a lower elastic recoil is transported at a slower rate than mucus of low viscosity and high elastic recoil [1525, 1526]. In dogs, the higher the mucus elastic modulus, the lower the clearance rate [1527]. The mucociliary clearance rate is halved by a 10-fold elevation in mucus elastic modulus. In frog palate, the mucociliary clearance rate is maximal for a mucus storage modulus of 0.16 Pa and sharply decreases above and below this value [1528]. In human subjects, optimal mucus rheology for mucociliary transport is given by a viscosity of 12 Pa ⋅s [1529] and an elastic modulus of 0.4 to 0.8 Pa [1530].
Chronic bacterial infection of a compartment of the respiratory tract that is often associated with tumors or foreign bodies usually leads to obstruction. However, airway obstruction is generally caused by mucus plugs that follow mucus adhesion and formation of mucus plaques.
When the mucus layer is too thick and clearance by the cilia is hindered, clearance by coughing takes over. In healthy subjects, coughing is less efficient than by mucociliary clearance, but not in patients with airway diseases.
12.3 Mucus Delivery
Mucus is secreted by secretory cells of the respiratory epithelium. Mucus is released by exocytosis in respiratory conduits down to terminal bronchioles. The mucus extends over the tops of the cilia in a layer of thickness of about 5 μm. Mucus has also been observed to exist as droplets, flakes, and plaques in airways. Droplets (size 4 μm) can aggregate into flakes (size 10–70 μ), and then plaques, but does not necessarily form a complete mucus blanket (mucus rafts). Nasal mucus has a thickness greater than 200 μm.
Mucus is secreted in healthy upper airways primarily by submucosal glands. It contains defense molecules mixed with mucins, electrolytes, and water. Mucus traps pathogens, impedes their proliferation using molecular (e.g., lysozyme) and cellular (e.g., neutrophils and macrophages) defenses, and sweeps them out of airways using ciliary beats.
12.3.1 Secretory Cells
Mucous and serous secretions form the protective airway surface fluid. Airway mucus is secreted by mucous cells, goblet cells of the respiratory epithelium and mucous cells of the submucosal glands. In large airways, the major part of respiratory mucus is produced by submucosal glands, whereas epithelial goblet cells yield a smaller contribution.
The secretory cell population is usually defined by 3 cell types according to their microscopical features — non-ciliated bronchiolar Clara, goblet, and serous cells. Serous and goblet cells reside principally in trachea and bronchi. Secretory cells of airways are classically identified according to morphological characteristics, such as the density and type of secretory granules and the proportion of smooth to rough endoplasmic reticulum. However, secretory cells are characterized by a great phenotype (structural, molecular, and functional) changeability.
Secretoglobins (ScGb1a1–ScGb1a2, ScGb1c1, ScGb1d1–ScGb1d2, and ScGb1d4, ScGb2a1, and ScGb3a1–ScGb3a2) that are expressed in secretory epithelia can serve as cell markers. Secretoglobin-1A1 (ScGb1a1), or Clara cell secretory protein (CCSP),3 is synthesized in cells that also produce mucins. Secretaglobin-3A1 is, like mucins, expressed selectively in secretory cells of large bronchi in humans [1532]. On the other hand, secretaglobin-3A2 is an early molecular marker for bronchiolar Clara cells.
12.3.1.1 Goblet Cell
Airwaygoblet cells are cylindrical-shaped, glandular, simple columnar epithelial cells. They use both apocrine (decapitation secretion, the apical portion of the secretory cell of the gland pinching off and entering the lumen) and merocrine (exocytosis, i.e., membrane-bound vesicle-mediated secretion through the plasma membrane in the lumen of an epithelial-walled duct) processes for secretion. They quickly ( < 100 ms) secrete mucins that covers airway lumen surface with a protective mucus that is exposed to more or less polluted inhaled air. Numerous goblet cells (density 3–5 × 104 /mm3) reside in the respiratory epithelium. Serous and Clara cells in small airways can transform into goblet cells.
Their apical secretory vesicles are discharged into the airway lumen to form a mucus layer over the epithelial surface. Peripheral granules in goblet cells produce the continuous baseline mucus, whereas core granules are secreted in response to stimuli [1531]. Goblet cells contain sialoglycoproteins or a mixture of sialo- and sulfoglycoproteins.
Secretory cells undergometaplasia, i.e., a change in phenotype.4Interleukin-13 causes goblet cell metaplasia via production and subsequent activation ofepidermal growth factor receptor. Interleukin-13 provokes transcrition of the SCGB1A1 gene in epithelial non-ciliated cells, more precisely, in non-granulated secretory and goblet cells [1533]. This change in phenotype does not depend upon proliferation; it is restricted to ScGb1a1 + cells of the tracheobronchial tree, but not ScGb1a1 + cells of bronchioles [1534].
Secretion of oligomeric mucins from airway goblet cells is regulated primarily by ATP and UTP nucleotides that activate Gq-coupledP2Y2 receptors, thereby triggering the PLC–IP3–Ca2 + and PLC–DAG–PKC intracellular cascades [1535]. These pathways coordinate actin cytoskeleton remodeling, thus enabling interaction of mucin secretory granules with apical membrane exocytic docking sites [1535].
Goblet and ciliated cells are indeed characterized by an apical distribution of β- and γ-actin. In goblet cells, actin forms a cortical sheet between granules and the apical plasma membrane that ruptures under ATP and UTP stimulation.Myristoylated alanine-rich C kinase substrate (MARCKS), a PKC-activated actin–plasma membrane-tethering protein, is phosphorylated upon nucleotide stimulation and translocates to the core cytosol [1535]. In addition,scinderin, or adseverin, a Ca2 + -activated actin filament severing and capping protein in human airway cells, once it is activated by Ca2 + influx, can disassemble actin filaments at sites of exocytosis and permit mucin secretion. Calcium ion contributes to the regulation of the final steps of exocytosis in goblet cells, as it interacts with rabphilin homolog, Uncoordinated-13 homolog Unc13b (or MUnc13),5 and synaptotagmin.
12.3.1.2 Submucosal Glands
In healthy humans, submucosal glands (thickness 0.15 mm for airway wall thickness 0.64 mm [mean gland–wall ratio range 0.14–0.36] [1536]) are estimated to provide most of the upper airway mucus. About half of the amount of intracellular mucins is stored in submucosal glands. Submucosal glands secrete about 40 times more mucus than secretory epithelial cells.
The body of the gland is located between the spiral bands ofsmooth muscle and cartilage plates. Tracheobronchial submucosal glands are tubular branching structures. Each gland contains multiple tubules that merge in a collecting duct.
Submucosal glands are only found in cartilagous-walled airways (upper aiways, trachea, and large bronchi) between the epithelium and cartilage. They abound down to about generation 10 (airway lumen caliber 1–2 mm) [1537]. Density of submucosal glands decays with increasing distance from the pharynx, whereas that of brush and intermediate cells rises.
In submucosal glands after transplantion in human patients with diseases other than cystic fibrosis, the secretion rate per gland upon stimulation byvasoactive intestinal peptide6 in tracheal and bronchial glands was 1.0 ± 0.2 and 1.1 ± 0.4 nl/mn, respectively [1538]. The volume of secretion depends on theNa + –K + –2Cl − cotransporter and onHCO3 − ion.
Submucosal glands are lined with 2 cell types, proximal mucous and distal serous cells. Mucous and serous cells constitute approximately 60 and 40% of the gland volume, respectively [1538]. Mucous and serous cells secrete a viscoelastic gel and a lubricating fluid (water and electrolytes) that bathes epithelial cilia, respectively.
At their distal ends (acini), serous cells secrete proteoglycans and numerous antimicrobial, anti-inflammatory, and anti-oxidant proteins [1538]. The serous fluid actually contains lysozyme, which breaks down cell walls and lactoferrin, which limits iron availability to bacteria, immunoglobulin-A, in addition to transferrin, albumin, Muc7, serum leukocyte protease inhibitor, and surfactant protein-A [1531, 1538]. Serous fluid washes proximal mucous cells that secrete mucins. Mucous cells contain acidic glycoproteins, whereas serous ceils produce mainly neutral glycoproteins.
Cystic fibrosis transmembrane regulator localizes primarily to serous cells. Elevation of cytosolic Ca2 + level is supposed to activate secretion of fluid, ions (Na + , Cl − , and HCO3 − ), mucins, and other proteins from both serous and mucous cells and increase in cytosolic cAMP level to stimulate serous cells and mucin, but not fluid secretion, from mucous cells [1538].
Each gland with serous acini and tubules followed by mucous tubules is connected to the airway lumen (gland opening density ∼ 1/mm2) by an upstream non-ciliated collecting duct and a downstream narrower ciliated duct that propels secretions outward.
The ciliated duct may have an absorptive function, as secretions from bronchial submucosal and nasal glands contain significantly less ions than the bath [1538]. In addition, they may acidify secretions usingH + –K + ATPase.
Collecting duct (length ∼ 1 mm) runs obliquely to the wall lumen. Its caliber rises along its length. Duct-lining non-ciliated cells may control ion and water content of gland secretion, as they are surrounded by abundant blood capillaries in opposition to the ciliated duct to which it connects, which is covered by a duct-lining, ciliated, mucus-secreting epithelium.
Both cholinergic and adrenergic innervations of submucosal glands exist. However, responsiveness (affinity) of serous and mucous cells to α- and β-adrenergic transmitters differs. In addition, serous cells receive a greater number of both nerve inputs (overall ratio of cholinergic to adrenergic innervation 9:1).
12.3.1.3 Clara Cells
Mucins are secreted not only by goblet cells and submucosal glands, but alsoClara cells. Clara cells are the most abundant secretory cell type in distal airways of the human lung.
Clara cell produces high levels of cytochrome-P450. It secretes the lipoprotein secretoglobin-1A1, or Clara cell secretory protein (CCSP). Whereas Muc5ac localizes to the surface epithelium, Muc5b lodges not only in submucosal glands, but also in Clara cells.
Clara cells can differentiate into mucous cells such as goblet cells [1539, 1540] (metaplasia). However, the molecular and functional features of the Clara cell are retained in mucous cells [1534]. Clara cells synthesize the regulated exocytic markerRab3d GTPase.7
Upon antigen and aerosolized ATP exposure, Clara cells rapidly secrete mucin. Extracellular purine and pyrimidine nucleotide triphosphates (adenosine [ATP] and uridine [UTP] 5′-triphosphate) stimulate mucin liberation by airway epithelial cells [1541]. The order of potency of nucleosides and nucleotides in stimulating mucin secretion in cultures of human tracheobronchial epithelial cells is the following:
ATP ∼ UTP > ADP ∼ UDP ≫ adenosine.
Agonists of nucleotide receptors are potent mucin secretagogues. Mucin is released in response to the G-protein–PLC–PKC pathway. However, only UTP can increase mucin gene (MUC5AC and MUC5B) transcription using the G-protein–MAPK axis. Therefore, in differentiated airway epithelial cell cultures, ATP causes mucin secretion and UTP both mucin synthesis and secretion.
The exocytic priming protein MUnc13-2, or Uncoordinated-13 homolog Unc13b, is required in the regulated, baseline secretory pathway of Muc5b in Clara cells [1542]. On the other hand, MUnc13-4, or Unc13d, a Rab27 effector, triggers ATP and UTP nucleotide-regulated release of accumulated mucins in secretory granules.
12.3.1.4 Nervous Control
The lungs and pleura are innervated by the mixed, anterior and posterior, pulmonary plexi that containvagal (parasympathetic) andsympathetic nerves. The pulmonary parasympathetic and sympathetic innervations originate from thoracic plexi. The pulmonary nerve plexus lies behind each hilum, receiving fibers from both vagi and thoracic T1 to T4 ganglia of the sympathetic trunk. Each vagus contains sensory afferents from airways and bronchoconstrictor and secretomotor efferents.
The main pulmonary nerves enter the lungs at their respective hila and then accompany bronchi and associated pulmonary arteries and veins. Each lobar bronchus has 4 to 5 nerves around it. Peribronchial nerves emit thick and thin fibers that enter the bronchial wall [1543]. Intrabronchial nerves give rise to smaller branches and bronchial ganglia.
Most airway mucus is produced by submucosal glands in response to neural signals [1544]. Various secretory chemical messengers synthesized in the neuroendocrine system enable short-range (neurotransmitters and paracrine regulators) and long-range (circulating hormones) communications.
Lung Innervation
The lung is supplied by motor nerves of the sympathetic and parasympathetic compartments of the autonomic nervous system and by sensory nerves that originate primarily from sensory ganglia of the vagus nerve, but also arise from dorsal root ganglia (Table 12.1) [1544].
Table 12.1
Sensory and preganglionic and postganglionic sympathetic and parasympathetic nerves and peptidergic innervation of the respiratory tract (Source: [1544]; CGRP: calcitonin gene-related peptide; NAd: noradrenaline; NPY: neuropeptide-Y; VIP: vasoactive intestinal peptide). Sensory nerves, which have cell bodies mainly in the vagal ganglia, especially the inferior ganglion of vagus nerve (or nodose ganglion), project axons into the pulmonary blood vessels, bronchi, cartilage, and other structures. The parasympathetic preganglionic neurons (vagus nerve) originate from the brain stem (primarily the dorsal motor nucleus and nucleus ambiguus); the parasympathetic postganglionic neurons innervate the intrapulmonary ganglia in the vascular adventitia and airway submucosa. Neurons of airway ganglia produce acetylcholine (ACh), VIP, substance-P (SP), galanin, enkephalins, and nitric oxide (NO). Preganglionic sympathetic fibers of the lungs originate from the intermediolateral horn of spinal cord segments (T1–T5 column), postganglionic neurons from ganglia of the sympathetic chain (superior and stellate ganglia). VIP + and NPY + neurons of sympathetic chain ganglia project to airways.
Released messengers | |
---|---|
Nerve type | Targets |
Parasympathetic nerves (vagus nerve) | |
Sensory nerves | CGRP, SP |
Preganglionic nerves | ACh |
Postganglionic nerves | ACh, NO, SP, VIP |
Respiratory epithelium, airway smooth muscle, | |
glands, bronchial arteries | |
Sympathetic nerves | |
Preganglionic nerves | ACh |
Postganglionic nerves | NAd, NPY, VIP |
Respiratory epithelium, airway smooth muscle, | |
glands, bronchial arteries |
Pulmonary plexi are located anterior and posterior to the lung roots, close to lung hila, which are constituted by stem bronchi, pulmonary arteries and veins, bronchial arteries and veins, and lymphatic vessels with bronchial lymph glands enclosed by a reflection of the pleura.
Pulmonary plexi innervatesmooth muscles of airways andblood vessels, and glands of the bronchial tree. Inner peribronchial nerve plexi reside in the lamina propria under the respiratory epithelium. They feed the bronchial smooth muscle and glands. Large bronchi possess peribronchial and subepithelial nervous plexi with intraepithelial nerve terminals. In bronchioles, the inner and outer nerve plexi merge to form a peribronchiolar plexus.
Postganglionic parasympathetic efferent nerves cause bronchoconstriction, vasodilation; and bronchial gland secretion. Parasympathetic afferent nerves are sensory fibers of the respiratory epithelium (stretch sensor) that ascend via the vagus nerves. Stretch receptors lodge in smooth muscles of bronchial walls, most being slowly adapting (firing with sustained stimulation), but others are rapidly adapting (transiently firing). Juxtapulmonary (J) receptors localize to alveolar and bronchial walls are connected to unmyelinated (C-fiber) or myelinated afferent nerves of vagus nerves. Postganglionic, adrenergic, sympathetic efferent nerves are bronchodilators, vasoconstrictors, and inhibitors of the glandular secretion.
The parasympathetic nervous system uses not only acetylcholine as a neurotransmitter, but also peptides. Stimulated preganglionic nerves (cell bodies in the medulla oblongata and nucleus ambiguus) release acetylcholine in the ganglion that targets nicotinic receptors of postganglionic neurons. The latter then releasesacetylcholine that activate muscarinic receptors of target cells.
Acetylcholine is also the principal neurotransmitter in all autonomic ganglia. In the autonomic nervous system, acetylcholine is actually released by all pre- and post-ganglionic parasympathetic neurons, all preganglionic sympathetic neurons, and some postganglionic sympathetic fibers.
The sympathetic innervation consists of cell bodies in the lateral horn of the spinal cord (intermediolateral cell columns from T1 to L3). These cell bodies belong to general visceral efferent (GVE) neurons and preganglionic neurons. The sympathetic trunks (a paired bundle of nerve fibers laterally along the spine column from the base of the skull to the coccyx) generate the paravertebral ganglia, or ganglia of the sympathetic trunk. Prevertebral ganglia (collateral or preaortic ganglia) localize between the sympathetic chain and supplied abdominal and pelvic organs. These ganglia give rise to postganglionic neurons. In fact, cardiopulmonary, thoracic splanchnic, sympathetic nerves that originate from the paravertebral ganglia contain noradrenergic postganglionic efferent and visceral afferent (sensory) fibers (general visceral afferent [GVA] neurons).Noradrenaline released from postganglionic sympathetic neurons participates to the control of airway behavior.
Neuropeptides
Neuropeptides contribute to regulate the body’s functions, in particular the body’s respiration, as: (1) para- and autocrine messengers; (2) neurohormones, which are produced by central neurons and reach their target cells of the nervous system via the blood circulation; (3) circulating hormones, which target remote cells; and (4) neurotransmitters and neuromodulators, which are released from nerve endings to act locally on nearby cell. A given neuron can manufactures one or more peptide hormones together with one or more neurotransmitters.
Numerous neuropeptides can be identified in the lung or influence its function. These neuropeptides are synthesized in neurons and released from nerve terminals. They encompass vasoactive intestinal peptide, bombesin, gastrin-releasing peptide (GRP), calcitonin gene-related peptide (CGRP), which are produced by neuroendocrine cells, in addition to serotonin [1544]. Calcitonin, endothelins, and angiotensin-converting enzyme, which forms angiotensin and degrades bradykinin, localize mainly to the endothelium.
In humans, the VIP/secretin family of brain–gut endocrine peptides include 10 members. In addition to vasoactive intestinal peptide and secretin, a hormone that controls water homeostasis throughout the body, this family encompasses adenylate cyclase-activating polypeptide AdCyAP1, or pituitary adenylate cyclase-activating peptide (PACAP), which may act as a sensory neuropeptide,8 PACAP-related peptide (PRP), peptide histidine methionine (PHM), glucagon, glucagon-like peptides GLP1 and GLP2,9 glucose-dependent insulinotropic peptide (GIP), and growth hormone-releasing hormone (GHRH).10 Vasoactive intestinal peptide is coproduced with peptide histidine methionine. Vasoactive intestinal peptide modulates cholinergic transmission in airways. In addition, the coexistence of VIP and substance-P in cholinergic nerves of airways yields a balance between the bronchoconstrictory, pro-inflammatory substance-P and the bronchiorelaxant, anti-inflammatory VIP [1544].11 Moreover, VIP andNO cooperate to relaxing airway andvascular smooth myocytes. Nitric oxide acts as a cotransmitter of non-adrenergic non-cholinergic (NANC) relaxation. Vasoactive intestinal peptide, directly or by interacting with other transmitters such as acetylcholine, stimulates airway secretion and ciliary mobility.
Pituitary adenylate cyclase-activating polypeptide AdCyAP1 can be observed in nerves aroundtracheal and bronchial smooth muscle, glands, and blood vessels [1544]. It coexists with the neuropeptidescalcitonin gene-related peptide andsubstance-P.
Tachykinin + nerves feed airways. Tachykinins are synthesized in the neuronal bodies of a subset of sensory neurons in dorsal root ganglia. Tachykinin + nerves distribute to the tracheobronchial epithelium, lamina propria, and smooth muscle, as well as to alveolar walls, systemic and pulmonary blood vessels, and airway ganglia [1544]. Inflammation is triggered by tachykinin release from nerve endings. Stimuli of tachykinin liberation in airways include bradykinin12 and various prostanoids.
The tachykinin family includessubstance-P,neurokinin-A (substance-K) and -B (neuromedin-K), and neuropeptide-K (neurokinin-K) and -γ. The TAC1 and TAC3 genes encode the tachykinin precursors preprotachykinin-A and -B. The TAC1 gene (or TAC2) generates multiple splice variants: substance-P, neurokinin-A, neuropeptide-K, and neuropeptide-γ. The TAC3 gene encodes neurokinin-B. The primary afferent neurons that produce tachykinins and innervate airways express the TAC1 gene [1544].
In airways, tachykinins coexist with calcitonin gene-related peptide, a potent vasodilator. Tachykinins pertain to the most powerful spasmogens onairway smooth myocytes. Substance-P and neurokinin-A target preferentially NK1 and NK2 receptors (Table 12.2).
Target cell | Tachykinin receptor types |
---|---|
Postganglionic nerves | NK1, NK2 (between-species variability) |
Tracheobronchial ganglia | NK1, NK2 |
Airway epithelial cell | NK1 |
Alveolar macrophage | NK2 |
Airway smooth myocyte | NK2 |
Seromucous glands | NK1 |
Pulmonary endothelial cell | NK1 |
Pulmonary smooth myocyte | NK2 |
Postcapillary venule | NK1 |
In human isolated airways, neurokinin-A is more potent than substance-P in causing long-lasting contraction [1544]. On the other hand, tachykinins provoke endothelium-dependent vasodilation of isolated precontracted pulmonary arteries as well as in perfused tracheal arteries of anesthetized dog, possibly preceded by transient vasoconstriction. They can thus have a mixed effects on the pulmonary and bronchial circulations. Tachykinins also raise airway mucosa microvascular permeability. Last, but not least, these powerful secretagogues stimulate ciliary beating in synergy with mucus secretion.
12.3.2 Mucus Release
Mucins are stored in a dehydrated form in secretory granules. Exocytosis is executed in 3 main steps: (1) motion of mucin granules to the apical plasma membrane; (2) fusion with the plasma membrane; and (3) opening onto the airway surface. Each calcium counterion within the granule is exchanged for 2 sodium ions in the extracellular space [1545]. Rapid secretion enables the formation of concentrated mucus that is resistant to dilution once the mucin network is formed.
Step 1 is regulated bymyristoylated alanine-rich C-kinase substrate (MARCKS) [1546]. Activated protein kinase-C phosphorylates MARCKS, causing MARCKS translocation from the plasma membrane to the cytoplasm. Agent MARCKS is then dephosphorylated by protein phosphatase PP2 that is activated by cGMP-dependent protein kinase PKG, itself stimulated by the NO–cGMP pathway. A cooperative interaction between protein kinases PKC and PKG is then required. Dephosphorylated cytoplasmic MARCKS associates with the contractile cytoskeleton (Vol. 1 – Chap. 6. Cell Cytoskeleton). Step 2 probably implicates a coordinated interaction between soluble Nethylmaleimide-sensitive factor receptors (SNARE), MUnc13-4,13 and small GTPaseRab.
12.3.3 Regulation of Mucus Secretion
Submucosal glands continuously secrete polymeric mucins at a low level. They can be further stimulated by adrenergic, cholinergic, and non-adrenergic, non-cholinergic nervous signals (Sect. 12.3.1.4). Therefore, 2 secretion mechanisms exist. The basal secretion at low level is unregulated. It is carried out by continuous movement of secretory granules driven by the cytoskeleton. The regulated secretion corresponds to granule exocytosis in response to extracellular stimuli to increase mucus secretion. Mucus secretion is elicited by irritations, such as dust and smoke.
The secretion of polymeric mucins is regulated separately from mucin production [1545]. Many mediators trigger mucin secretion, such as cholinergic agonists (also called parasympathometic agents), lipid mediators, oxidants, cytokines, neuropeptides, neutrophil elastase, ATP and UTP, etc. On the other hand,interleukin-4, -9, and -13 provoke mucin synthesis.
Muscarinic M3 receptors are detected onairway smooth myocytes as well as submucosal glands, whereas M l and M2 receptors are located inparasympathic ganglia and cholinergic nerves in lungs, respectively. Receptor M l is also observed in submucosal glands.
In humans,adrenergic nerves of airways could stimulate mucus secretion viaα- andβ-adrenoreceptors. β-Agonists stimulate ciliary beating in a dose-dependent fashion. A third component of the autonomic nervous system of lungs, the non-adrenergic, non-cholinergic nervous component represents the single inhibitory nervous mechanism in humans.Vasoactive intestinal peptide in conjunction with peptide histidine methamine serves as neurotransmitter. It not only increases fluid transport across the epithelium and mucin secretion, but also can inhibit resting secretion of mucus. It raises cAMP concentration in submucosal glands, respiratory epithelium, and airway smooth muscle.
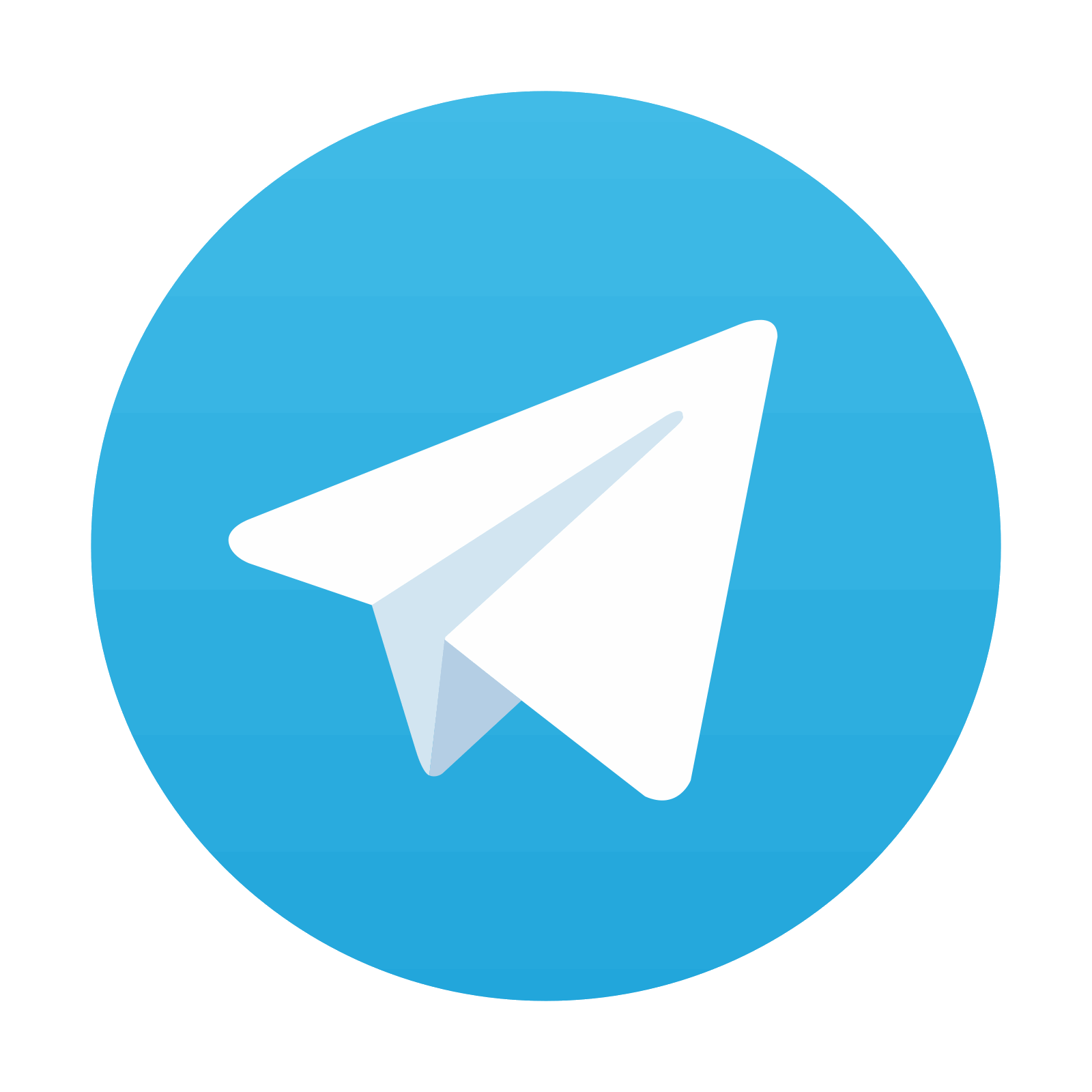
Stay updated, free articles. Join our Telegram channel
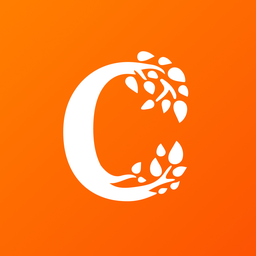
Full access? Get Clinical Tree
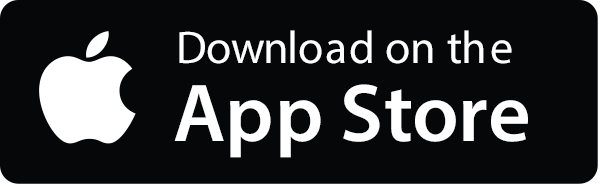
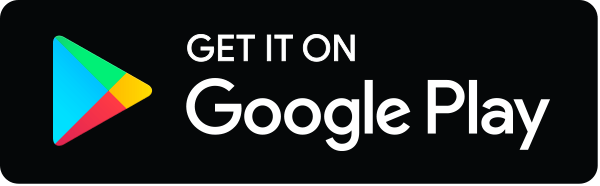