Chapter 2 Airway Management
Oxygenation and Ventilation
Upon completion of this chapter, you will be able to:
Anatomy of the Respiratory System
Upper Airway
The upper airway extends from the mouth and nose to the trachea (Figure 2-1). The upper airway functions as a passageway for gas flow; for filtering, warming, and humidifying the air; and for protecting the surfaces of the lower respiratory tract. The upper airway also functions in phonation and in the senses of smell and taste.
The oropharynx begins at the uvula, which is fleshy tissue that hangs down from the soft palate and into the posterior portion of the oral cavity. The posterior portion of the oral cavity opens into the oropharynx. The oropharynx extends to the upper rim of the epiglottis. The epiglottis is a small, leaf-shaped piece of cartilage located at the top of the larynx that prevents foreign material from entering the trachea during swallowing. The oropharynx functions in respiration and digestion. The anterior oropharynx opens into the oral cavity, which is comprised of the lips, cheeks, teeth, tongue, and hard and soft palates (Figure 2-2). The anterior roof of the oral cavity is formed by the maxillary bone and is called the hard palate. The posterior portion of the roof of the mouth is called the soft palate because it is made up of mucous membrane, muscular fibers, and mucous glands. The cheeks form the walls and the tongue dominates the floor of the oral cavity. Located on the lateral walls of the oropharynx are a pair of palatine tonsils that can cause a partial airway obstruction if they become excessively swollen. The space (or “pocket”) between the base of the tongue and the epiglottis is called the vallecula. When performing orotracheal intubation, the epiglottis is lifted out of the way to visualize the area during the passage of the tracheal tube between the vocal cords. The vallecula is an important anatomical landmark to identify when intubating a patient with the use of a curved laryngoscope blade.
The larynx (voice box) connects the pharynx to the trachea at the level of the cervical vertebrae (Figure 2-3). It conducts air between the pharynx and the lungs; it prevents food and foreign substances from entering the trachea; and it houses the vocal cords, which are involved in speech production. The larynx is a tubular structure made up of muscles, ligaments, and nine cartilages (Figure 2-4). The thyroid cartilage (Adam’s apple) is the largest and most superior cartilage of the larynx. It is more pronounced in adult males than adult females. The thyroid gland lies over the outer surface of the thyroid cartilage. The pyramid-shaped arytenoid cartilages of the larynx serve as a point of attachment for the vocal cords. The arytenoid cartilages often serve as an important landmark during intubation.
Lower Airway
The lower airway extends from the larynx to the alveoli, and it functions in the exchange of oxygen and carbon dioxide. Air moves from the larynx through the glottic opening and into the trachea. The adult trachea is about 12 cm in length and has an inner diameter of about 2 cm. It divides (bifurcates) into two separate tubes called the left and right primary bronchi (Figure 2-5). The point where the trachea divides into the right and left primary bronchi is called the carina. The right bronchus serves three lobes of the lung and the left bronchus serves two.
The right primary bronchus is straighter or less angled than the left, because the heart occupies space in the left chest cavity. These angles become important for several reasons. First, when intubating, if the tip of the tracheal tube is inserted too deeply, it will most likely lie within the right primary bronchus. When this occurs, your assessment of tube placement when auscultating the chest will reveal good lung sounds on the right and diminished or absent sounds over the left chest. In this situation, withdraw the tracheal tube a few centimeters and then reassess. In addition, foreign bodies tend to make their way into the right primary bronchus more often than they do into the left.1
The primary bronchi branch into narrowing secondary and tertiary bronchi, which then branch into bronchioles (Figure 2-6). As the bronchi continue to divide into the lung tissue and become smaller passageways, they become bronchioles. Bronchioles are composed entirely of smooth muscle that is supported by connective tissue. Bronchioles are responsible for regulating the flow of air to the alveoli. The stimulation of beta-2 receptor sites in the bronchioles results in relaxation of bronchial smooth muscle. After multiple subdivisions, the bronchioles divide into tiny tubes called alveolar ducts, where gas exchange first becomes possible. These ducts end in alveoli, which are tiny, hollow air sacs. Each lung of an average adult contains about 300 million alveoli, and each alveolus is surrounded by a pulmonary capillary. Oxygen diffuses through the thin walls of the alveoli to the capillaries, and carbon dioxide diffuses from the capillaries to the alveoli.
Lung Volumes and Capacities
Lung Volumes
During normal, quiet breathing, an adult male moves an average of 500 mL (5-7 mL/kg)2,3 of air into and out of the respiratory tract; this amount is called the tidal volume (Figure 2-7). Tidal volume can be indirectly evaluated by observing the rise and fall of a patient’s chest.
During forceful breathing (such as during and after heavy exercise), an additional 3000 mL2,3 can be inspired (this is the inspiratory reserve volume), and an extra 1200 mL2,3 or so can be expired (this is the expiratory reserve volume).
Residual volume is the amount of air that remains in the respiratory tract after a forceful expiration. In the average adult the residual volume is 1200 mL.2,3
Lung Capacities
Lung capacities include two or more lung volumes. The inspiratory capacity is comprised of the tidal volume plus the inspiratory reserve volume, and it is approximately 3500 mL (500 mL + 3000 mL).2,3
The functional residual capacity is composed of the expiratory reserve volume plus the residual volume, which is approximately 2400 mL (1200 mL + 1200 mL).2,3 Functional residual capacity is the volume of air that remains in the lungs at the end of a normal expiration.
The vital capacity is the amount of air that can be forcibly expired after a maximal inspiration. It is composed of the inspiratory capacity plus the expiratory reserve volume, and it is about 4700 mL (3500 mL + 1200 mL).2,3 Its value increases with body size, male gender, and physical conditioning, and it decreases with age. Vital capacity reflects the largest volume of air that can be moved in and out during ventilation.
The total lung capacity includes all of the lung volumes: It is the vital capacity plus the residual volume, which is about 5900 mL (4700 mL + 1200 mL).2,3
Devices for Assessing Oxygenation and Ventilation
Pulse Oximetry
[Objective 1]
Oxygenation is the process of getting oxygen into the body and to its tissues for metabolism. Pulse oximetry is a noninvasive method of measuring oxygen saturation of functional hemoglobin. A pulse oximeter, which is commonly called a pulse ox, is a small instrument with a light sensor. The sensor is typically applied to a finger, but the forehead, an earlobe, or a toe can also be used with the selection of a sensor that is appropriate for the chosen site. For example, an adhesive or clip-on sensor can be used for a finger, while a forehead sensor is usually adhesive. The placement of a sensor in a central location such as the earlobe may reflect the most rapid oximeter response (i.e., within about 10 seconds) to a drop in the saturation of peripheral oxygen (SpO2). With finger placement, the response delay may take 30 to 60 seconds, and with toe placement, the delay may take up to 90 seconds.5
Pulse oximetry sensors may be disposable or reusable. Disposable foam-wrap or adhesive sensors are recommended when the patient is active, when monitoring continuously for more than 10 minutes, and when a risk for cross-contamination with microbial pathogens exists.6 When using a disposable sensor, the American Association of Critical-Care Nurses (AACN) recommends assessing the site every 2 to 4 hours and replacing the sensor every 24 hours.7 Assess the site for decreased temperature, decreased peripheral pulse, cyanosis, and tissue integrity. Reusable clip-on sensors are generally used when spot-checking oximetry values, when monitoring continuously for less than 10 minutes, and when monitoring patients who are immobile (Figure 2-8). When a reusable sensor is applied, the AACN recommends assessing the site every 2 hours and changing the site every 4 hours.7
The instrument sensor emits two light frequencies: one is a red beam that is the approximate color of oxygenated hemoglobin, and the other is an infrared beam that is the approximate wavelength of deoxygenated hemoglobin (Figure 2-9). By measuring the absorption of the two frequencies, the oximeter quickly and accurately calculates the percentage of hemoglobin that is saturated with oxygen in a pulsating capillary bed. This calculation is called the saturation of peripheral oxygen or SpO2. The oximeter displays this value as a percentage and the patient’s pulse rate on its screen. The SpO2 is a generally reliable indicator of the status of the partial pressure of oxygen in the arterial blood or PaO2.1 A healthy adult who is breathing room air at sea level generally has an SpO2 in the range of 96% to 100%. Patients with SpO2 values that are significantly lower than normal are likely to be hypoxic.
You Should Know
After hyperoxygenation, the oxyhemoglobin saturation detected by pulse oximetry may not decline for as long as 3 minutes, even when ventilation is ineffective.8–10
Possible indications for continuous pulse oximetry monitoring include the following:
Pulse oximetry may be inaccurate in situations that involve poor capillary blood flow, an abnormal hemoglobin concentration, or an abnormal shape of the hemoglobin molecule. Examples of conditions that may give misleading results are listed in Box 2-1.
BOX 2-1 Factors Affecting the Accuracy of Pulse Oximetry Readings
Carbon Dioxide Monitoring
[Objective 1]
Carbon dioxide is produced during cellular metabolism, carried to the lungs by the circulatory system, and excreted by the lungs during ventilation. Capnography is the continuous analysis and recording of CO2 concentrations in respiratory gases. Capnography-related terms appear in Table 2-1. Capnography provides healthcare professionals with breath-to-breath patient information, thereby enabling the early recognition of hypoventilation, apnea, or airway obstruction and thus preventing hypoxic episodes. The monitoring of exhaled carbon dioxide with either capnometry or capnography can detect changes in metabolism, circulation, respiration, the airway, or respiratory system.
TABLE 2-1 Capnography-Related Terms
Term | Description |
---|---|
Capnography | Continuous analysis and recording of CO2 concentrations in respiratory gases |
Output displayed as a waveform | |
Graphic display of the CO2 concentration vs. time during a respiratory cycle | |
CO2 concentration may also be plotted vs. expiratory volume | |
Most reliable method for confirmation and monitoring of tracheal tube placement | |
Capnometer | Device used to measure the concentration of CO2 at the end of exhalation |
Capnometry | A numeric reading of exhaled CO2 concentrations without a continuous written record or waveform |
Output is a numerical value | |
Numeric display of CO2 on a monitor | |
Capnograph | A device that provides a numeric reading of exhaled CO2 concentrations and a waveform (tracing) |
Colorimetric EtCO2 detector | A device that provides CO2 readings by chemical reaction on pH-sensitive litmus paper housed in the detector |
The presence of CO2 (evidenced by a color change on the colorimetric device) suggests tracheal tube placement | |
EtCO2 detector | A capnometer that provides a noninvasive estimate of alveolar ventilation, the concentration of exhaled CO2 from the lungs, and arterial carbon dioxide content |
Qualitative EtCO2 monitor | A device that uses a light to indicate the presence of EtCO2 |
Exhaled carbon dioxide detection devices are used in conjunction with the history and clinical assessment of the patient, which may include mental status, lung sounds, pulse rate, and skin color. An example of a combination handheld capnograph and pulse oximeter is shown in Figure 2-10. Examples of situations in which exhaled CO2 monitoring is commonly used include the following:
Alveolar CO2 and arterial CO2 (PaCO2) values are closely related in patients with normal cardiopulmonary function, and they usually range between 35 and 45 mm Hg. In patients with normal lung and cardiac function, end-tidal carbon dioxide (EtCO2) is usually 2 to 5 mm Hg less than the PaCO2 because of the contribution of physiologic dead space gas to the end-tidal gases.13 The normal values for EtCO2 range between 33 mm Hg and 43 mm Hg. This is dependent upon adequate ventilation and adequate perfusion: a change in either factor will increase or decrease the amount of exhaled CO2.
Capnography devices function through infrared technology. In addition to providing quantitative data as do digital capnometers, they also provide information about air movement in and out of the lungs with a graphical waveform. The process of CO2 elimination produces a characteristic waveform called the capnogram. An example of a normal capnogram is shown in Figure 2-11. A and B of the waveform show the baseline and represent the beginning of expiration, when air from the anatomic dead space is being exhaled. Because this air contains undetectable amounts of CO2 there is no movement of the wave from the baseline. B and C (expiratory upstroke) reflect the rapid upstroke of the curve and represent the transition from inspiration to expiration and the mixing of dead space and alveolar gas. C and D (expiratory plateau) show the alveolar plateau, which represents alveolar gas that is rich in carbon dioxide passing the sensor. The plateau tends to slope gently upward with the uneven emptying of the alveoli. D and E (inspiratory downstroke) demonstrate a rapid, sharp downstroke that represents the change to the inspiratory portion of the respiratory cycle. The downstroke is a nearly vertical drop to the baseline that reflects the rapid decrease in the levels of carbon dioxide passing the sensor. D reflects the point at which expiration ends and inspiration begins, and it is the end-tidal CO2 (EtCO2) value. It is this number that is reported by most capnometers. The space that occurs between waveforms is the result of the pause between ventilations. Changes in the EtCO2 generally result from changes in perfusion or ventilation rates. Changes in the morphology of the waveform indicate a change in air movement through the lower airways caused by alterations in metabolism, circulation, ventilation, or equipment function. During cardiac arrest, waveform capnography appears to demonstrate high sensitivity and specificity for proper tracheal tube placement.
A colorimetric capnometer functions through a pH change that occurs with the breath of a patient (Figure 2-12). The patient’s breath causes a chemical reaction on pH-sensitive litmus paper housed in the detector. The capnometer is placed between a tracheal tube or advanced airway device and a ventilation device (Figure 2-13). The presence of CO2, which is evidenced by a color change on the colorimetric device, suggests placement of the tube in the trachea. A colorimetric capnometer is qualitative in that it simply shows the presence of CO2. It has no ability to provide an actual CO2 reading or to indicate the presence of hypercarbia, and it provides no opportunity for ongoing monitoring to ensure that the tube remains in the trachea. A lack of CO2 (i.e., no color change) suggests tube placement in the esophagus, particularly in patients with a perfusing rhythm (not in cardiac arrest).
Some manufacturers of colorimetric capnometers recommend ventilating the patient at least six times before attempting to use an exhaled CO2 detector to assess tracheal tube placement. The rationale for this action is to quickly wash out any retained CO2 that is present in the stomach or esophagus as a result of bag-mask ventilation. CO2 that is detected after six positive pressure ventilations can be presumed to be from the lungs.14,15
In animals, false-positive results (i.e., CO2 is detected despite tube placement in the esophagus) have been reported when large amounts of carbonated beverages were ingested before a cardiac arrest.15 False-negative results (i.e., a lack of CO2 detection despite tube placement in the trachea) may occur because of poor pulmonary blood flow, such as in patients with cardiac arrest, severe airway obstruction (e.g., status asthmaticus), pulmonary edema, or significant pulmonary embolus.16 Colorimetric capnometers are susceptible to inaccurate results as result of the age of the paper and exposure of the paper to the environment. A colorimetric capnometer may not change color if the paper is contaminated with patient secretions (e.g., vomitus) or acidic drugs (e.g., tracheally administered epinephrine).17 When CO2 is not detected, an alternative method should be used to confirm tracheal tube placement, such as direct visualization or the use of an esophageal detector device.18
Oxygen Delivery Devices
The fraction of inspired gas that is oxygen is abbreviated as FIO2 and is often expressed as a percentage. Supplemental oxygen administration is indicated if the patient is cyanotic; if he or she is having difficulty breathing or having obvious signs of heart failure or shock, or if his or her oxygen saturation level declines to less than 94%. Titrate oxygen therapy to maintain the patient’s SpO2 at 94% or more.19
Nasal Cannula
[Objective 2]
A nasal cannula, which is also called nasal prongs, is a piece of plastic tubing with two soft prongs that project from the tubing. The prongs are inserted into the patient’s nostrils and the tubing is then secured to the patient’s face (Figure 2-14). Oxygen flows from the cannula into the patient’s nasopharynx, which acts as an anatomic reservoir.
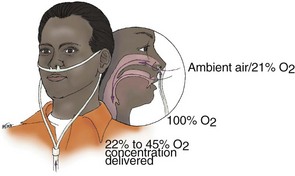
Figure 2-14 At flow rates of 0.25 to 8 L/min, a nasal cannula can deliver an oxygen concentration of 22% to 45%.
Although the actual inspired oxygen concentration depends on the patient’s ventilatory rate and depth, a nasal cannula can deliver oxygen concentrations of about 22% to 45% at 0.25 to 8 L/min flow.20,21 Flow rates of more than 6 L/min do not enhance delivered oxygen concentration; they dry the mucous membranes of the nasal cavity, and they often cause discomfort (e.g., headaches). Advantages and disadvantages of using a nasal cannula are shown in Box 2-2.
Simple Face Mask
[Objective 2]
A simple face mask, which is also called a standard mask, is a plastic reservoir that has been designed to fit over the nose and mouth of a spontaneously breathing patient. The mask is secured around the patient’s head by means of an elastic strap. The internal capacity of the mask produces a reservoir effect. Small holes on each side of the mask allow for the passage of inspired and expired air. Supplemental oxygen is delivered through a small-diameter tube connected to the base of the mask (Figure 2-15).
At 5 to 10 L/min, the simple face mask can deliver an inspired oxygen concentration of approximately 35% to 60%. The patient’s actual inspired oxygen concentration will vary, because the amount of air that mixes with supplemental oxygen is dependent on the patient’s inspiratory flow rate. Advantages and disadvantages of using a simple face mask are shown in Box 2-3.
Partial Rebreather Mask
[Objective 2]
A partial rebreather mask is similar to a simple face mask, but it has an attached oxygen-collecting device (i.e., reservoir) at the base of the mask that is filled before patient use. One hundred percent oxygen is delivered through oxygen tubing to the reservoir bag. The reservoir collects the oxygen and allows some of the patient’s exhaled air (i.e., an amount that is approximately equal to the volume of the patient’s anatomic dead space) to enter the reservoir bag and be reused (Figure 2-16).
The oxygen concentration of the patient’s exhaled air, in combination with the supply of 100% oxygen, allows for the use of oxygen flow rates that are lower than those that are necessary for a nonrebreather mask. Depending on the patient’s breathing pattern, the mask fit, and the oxygen flowmeter setting, oxygen concentrations of 35% to 60% can be delivered when an oxygen flow rate is used that prevents the reservoir bag from completely collapsing on inspiration (i.e., typically 6-10 L/min).20 Advantages and disadvantages of using a partial rebreather mask are shown in Box 2-4.
Nonrebreather Mask
[Objective 2]
A nonrebreather mask is the delivery device of choice when high concentrations of oxygen are needed for the spontaneously breathing patient. Depending on the patient’s breathing pattern, the fit of the mask, and the oxygen flowmeter setting, oxygen concentrations of 60% to 80%21 can be delivered when an oxygen flow rate (typically a minimum of 10 L/min) is used that prevents the reservoir bag from collapsing completely on inspiration. Inflate the reservoir bag with oxygen before placing the nonrebreather mask on the patient (Figure 2-17). Advantages and disadvantages of using a nonrebreather mask are shown in Box 2-5. A summary of oxygen percentages by device is shown in Table 2-2.
BOX 2-5 Nonrebreather Mask—Advantages and Disadvantages
• Higher oxygen concentration delivered than by nasal cannula, simple face mask, and partial rebreather mask |
TABLE 2-2 Oxygen Percentage Delivery by Device
Device | Approximate Inspired Oxygen Concentration | Liter Flow (Liters/Minute) |
---|---|---|
Nasal cannula | 22% to 45% | 0.25 to 8 |
Simple face mask | 35% to 60% | 5 to 10 |
Partial rebreather mask | 35% to 60% | Typically 6 to 10 to prevent bag collapse on inspiration |
Nonrebreather mask | 60% to 80% | Typically a minimum of 10 to prevent bag collapse on inspiration |
Manual Airway Maneuvers
An important consideration when determining which maneuver to perform is the possible presence of spinal trauma. Because manual maneuvers manipulate the cervical spine, a modification is required to minimize spinal movement and to maintain a neutral spinal position. When opening a patient’s airway, the fact that you are doing so means that your patient is unable to maintain his or her own airway. You must be prepared with additional equipment (e.g., airway adjuncts, a ventilation device) and anticipate the need for suction.1
Head Tilt–Chin Lift
[Objective 3]
The head tilt–chin lift is the preferred technique for opening the airway of an unresponsive patient without suspected cervical spine injury (Figure 2-18). Follow these steps to perform a head tilt–chin lift:
Jaw Thrust
Jaw Thrust without Head Tilt
[Objective 3]
The jaw thrust without head tilt maneuver (also called the modified jaw thrust) is the technique that is recommended for opening the airway when cervical spine injury is suspected (Figure 2-19). Perform the following for a jaw thrust without head tilt maneuver:
Manual airway maneuvers are summarized in Table 2-3.
TABLE 2-3 Manual Airway Maneuvers
Considerations | Head Tilt–Chin Lift | Jaw Thrust without Head Tilt |
---|---|---|
Indications | ||
Contraindications | ||
Advantages | ||
Disadvantages |
< div class='tao-gold-member'>
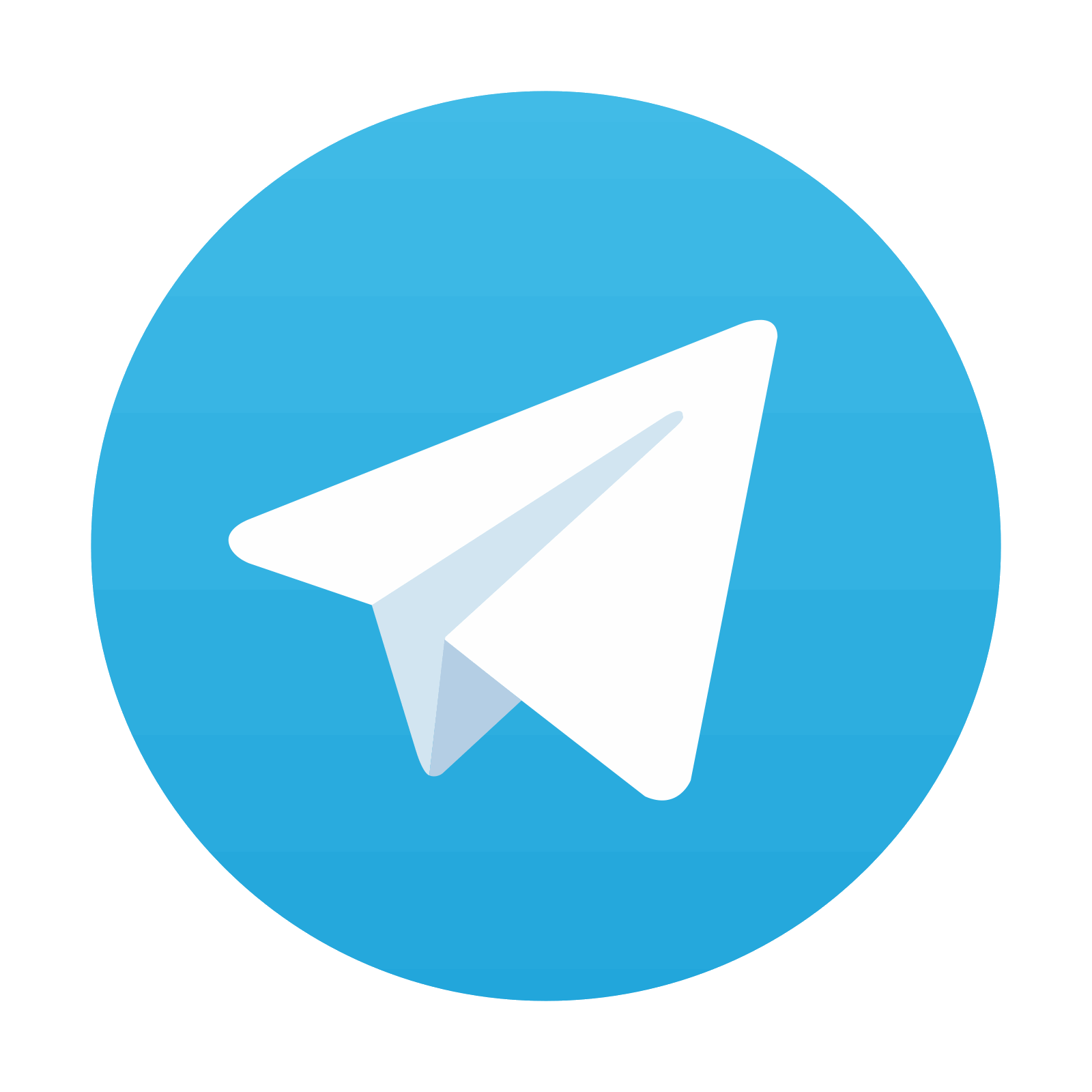
Stay updated, free articles. Join our Telegram channel
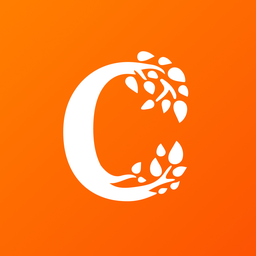
Full access? Get Clinical Tree
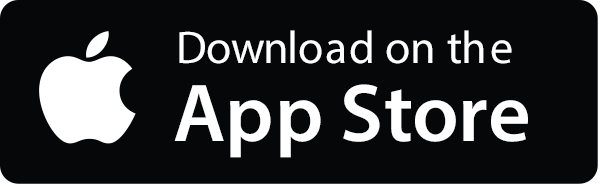
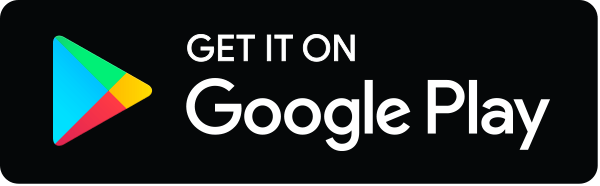