4 • blocking of the airway by secretions, e.g. in bronchitis • reduction in airways diameter due to contraction of smooth muscle in its walls or swelling due to inflammation, e.g. in asthma and bronchitis • collapse of the airways due to disruption of the supporting parenchyma, e.g. in emphysema, or changes in intrapleural pressure, as in cough. Our model from the previous chapter, which represents the respiratory system as a balloon inside a container like a syringe, is often used to illustrate the two components of intrapleural pressure mentioned above and illustrated in Figure 4.1. The plunger of the syringe represents the diaphragm, the walls of the syringe represent the chest wall, the balloon represents the alveoli, and the narrow tube represents the airways of the lung. 1. The changes in intrapleural pressure that bring about breathing can be superimposed on a small lung volume (the balloon being only slightly inflated) or on a large lung volume (the balloon very inflated). Understandably, to hold the balloon at a large volume requires greater effort, and this is what happens, for example, in asthma, where the patient breathes at an increased lung volume to keep his airways open. 2. Airflow only takes place from a region of high pressure to a region of low pressure along the airway. Therefore, at two times in each respiratory cycle (when inspiration has just ceased and is about to become expiration, or when expiration has just ceased and is about to become inspiration) there is no flow, and pressure is the same all the way from the lips to the alveoli. This fact can be used to measure dynamic lung compliance (see Fig. 3.17). It should also be noted that under conditions of normal breathing the pressure in the airways is much closer to atmospheric pressure than the pressure in the intrapleural space. 3. Recoil of the lungs produces a pressure which resists inspiration but assists expiration. This is analogous to pushing a car up a hill: the weight of the car represents recoil pressure and resists going up (inspiration). The weight of the car would, however, assist going down (expiration). This effect of helping expiration and hindering inspiration is seen in flow/volume loops used to test lung function. Flow in a long straight smooth tube becomes turbulent when Reynolds’ Number, which is calculated as 2rvd/η (r = radius of tube, v = velocity of flow, d = gas density, η = gas viscosity) exceeds 2000. Under turbulent conditions flow varies with the square root of the driving pressure, i.e. it is very much more difficult to produce the same flow when flow is turbulent (Fig. 4.2). where r is the radius of the tube, η is the viscosity of the gas, and l is the length of the tube. This relationship applies to gas flow in the long straight smooth tubes under stable conditions – hardly conditions that apply to the lungs. However, it can be roughly applied to breathing, and you may notice that the most important factor affecting airflow in this equation is the radius of the tube, which is raised to the fourth power (r4). This means that if you reduce the radius by half and keep everything else constant, the flow will be reduced to Laminar flow in a tube can be represented as a series of cylinders moving down the tube, with the central cylinder moving fastest. The outermost cylinder is stationary and is in fact a layer of the original gas in the tube left behind as the new gas moves forward, as shown in Figure 4.4. These apparently esoteric considerations have important consequences in respiratory medicine. For example, adequate ventilation of the alveoli of the lungs can be achieved with a surprisingly small tidal volume, provided a high enough frequency of ‘breathing’ is used. This phenomenon is seen in clinical conditions when high-frequency artificial ventilation of the lungs is used where we want to avoid movements of the chest wall – in trauma victims with a crushed chest, for example. The patient is successfully artificially ventilated with a tidal volume less than his or her anatomical dead space (see p. 64) and frequencies up to 50 Hz. A ‘spear’ of fresh air in the centre of the airways penetrates deeper into the lungs than might be expected and provides adequate ventilation. We have established that it takes a greater pressure to inflate the lungs than simply to hold them in a steady inflated state. This extra pressure is required to produce flow in the airways. We have also seen that the relationship between pressure and volume on slowly inflating and deflating the lungs is not a straight line, as you would get with a rubber balloon, but rather a loop, with a greater pressure needed to inflate the lungs to a given volume than that which exists at the same volume when they are being allowed to deflate (Fig. 4.3). The small dotted loop in Figure 4.3 was produced by inflating and deflating the lungs extremely slowly, a situation that is called pseudostatic. If we were to carry out this inflation and deflation at a normal breathing rate (12 times per minute, say) the loop would be wider (the solid line), with inflation pressures required for a given volume being greater than under the static conditions and deflation pressures being less. This situation arises because energy is used up to propel the air along the airways; the airways can be said to resist flow in a phenomenon known as airways resistance. This resistance during laminar flow can be thought of as the friction between the layers of air as they are pushed down the airway. Pushing a pile of typing paper or a pack of cards across a table gives a good idea of what is happening (Fig. 4.4). As its name implies, airways resistance is analogous to electrical resistance. To measure the electrical resistance of a length of wire you need to know two things, the potential difference (voltage) between the two ends of the wire, and the current flowing in the wire. Using reasonable currents (that do not overheat the wire) we find that the relationship between voltage (V) and current (I) is a straight line (Fig. 4.5). This is Ohm’s Law, and the slope of the line (V/I) is the resistance of the wire in ohms. The slope of the line is the airway resistance of the tube in kPa 1−1 s. Two important points arise from this apparently innocuous statement: 1. Airways resistance is a dynamic property and can only be measured when flow is taking place. 2. The figures used above to arrive at the figure of 0.2 kPa 1−1 apply equally well if flow is in an inspiratory or an expiratory direction: they are a property of the tube, not the direction of the flow. Airways resistance changes in disease and its measurement has been the focus of interest of doctors for many years. Measurement of airflow is not difficult and is usually accomplished using an instrument known as a pneumotachograph, which itself illustrates the principle of airways resistance. The pneumotachograph consists of a tube through which the subject breathes (Fig. 4.6). One way of getting round the problem of measuring the driving pressure is to use the fact that it is the change in alveolar pressure that brings about flow and stretches the lungs against recoil pressure. Consider the changes in intrapleural pressure and recoil pressure due to the elasticity of the lungs in a single respiratory cycle (Fig. 4.7). Subtract changes in recoil pressure from changes in intrapleural pressure and you are left with the changes in alveolar pressure that produce changes in airflow – the two variables we are interested in to measure resistance. This subtraction process can be done instantaneously electronically, and so we need to know the changes in recoil pressure, which can be worked out from lung compliance, and the changes in intrapleural pressure, which we obtain by measuring changes in the pressure inside the oesophagus passing through the intrapleural space. This consists of an airtight box in which the subject sits. It depends on the principle that the total quantity of air in the box (inside the patient’s lungs and around him in the box) is the same throughout the measurement – the box is airtight. The patient first pants against a closed shutter while the pressure changes in his mouth and the pressure changes in the box are measured simultaneously. The pressure changes at his mouth are assumed to be the same as those in the alveoli (by the same arguments as in the interrupter technique above). Pressure changes in the box while the patient is panting because he is compressing and decompressing the air in his lungs and therefore changing the volume of his chest in the closed box. (Consider him like the syringe in Figure 4.8: when he compresses the air in his chest he decompresses the air in the box, and when he decompresses the air in his chest he compresses the air in the box.) The pressure changes in the box are therefore related to pressure changes in the alveoli, and so by measuring box pressure change and flow while the patient breathes through a pneumotachograph, we can measure his airways resistance. Other uses of the whole-body plethysmograph are described in Chapter 11. Of the approximately half total airway resistance existing below the larynx 80% resides in the trachea and bronchi. This is difficult to reconcile with Poiseuilles’s law (p. 46, 156) that the airway resistance of a tube is proportional to the fourth power of its radius, as the trachea and main bronchi are the largest tubes in the bronchial tree. The explanation for this is that the number of airways doubles at each airway branching (each airway normally splits into two daughters), therefore the number (n) at each generation (g) (see p. 15) is n = g2, starting with the two main bronchi as generation 1 (Fig. 4.9). This rapid increase in numbers more than offsets the decrease in diameter of the individual members of each generation, and the total cross-sectional area increases dramatically. The bronchi extend from generation 1 to 16, with the small bronchi (generations 5–11; 3.5–1 mm diameter) not being directly attached to the lung parenchyma. They are supported against collapse by cartilage and the transmural pressure gradient, which it is now believed rarely reverses sufficiently to cause complete collapse of these airways.
AIRFLOW IN THE RESPIRATORY SYSTEM
Introduction
How airflow is brought about
The nature of airflow
The major determinant of flow – radius
.
Airways resistance and obstructive pulmonary disease
The clinical situation
The whole-body plethysmograph
Sites of airways resistance
AIRFLOW IN THE RESPIRATORY SYSTEM
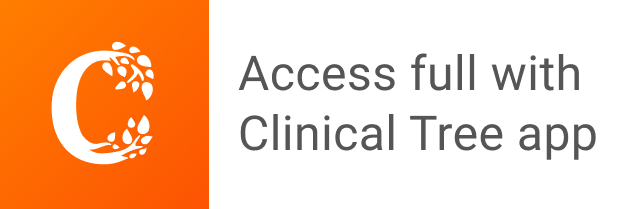