Perhaps one of the most vexing aspects of congenital cardiac disease is the current inability to explain its origin. Environmental causes have been invoked, and until recently only scant evidence had pointed towards a genetic component. Recent experimental data, combined with advances in human genetics, have now provided a clearer understanding of how some malformations may occur, and certainly have illuminated general concepts that are certain to apply to congenital cardiac disease in general. One of the most important developments has been the paradigm shift from grouping lesions based on clinical presentation, to understanding the anomalies based on their embryonic and genetic origins. Thus, it is now clear how an inherited mutation can result in a family where one individual has an interatrial communication, while another tetralogy of Fallot, and still be considered the same genetic defect. In this chapter, we will review the various aetiologies, environmental and genetic, with a constant eye towards the embryology of the heart, with the hope that by synthesising the current knowledge, we will provide a useful insight into the fundamental basis of congenital cardiac malformations.
EPIDEMIOLOGY OF HEART DISEASE: GENES VERSUS ENVIRONMENT
The study of the aetiology of congenital cardiac disease initially focused on epidemiological studies, which mainly incorporated the identification of factors that influence the incidence of the various lesions. This is in large part because familial inheritance is not obvious, and thus a tractable focus is environmental influence and assessment of heritability. These studies primarily led to the conclusion that there were multi-factorial influences. Several difficulties are apparent with these studies. First, intra-uterine mortality due to congenital cardiac disease is difficult to assess, and conversely, in addition to the nearly 1% of children with cardiac malformations, 1 an additional 1% to 2% of the population harbour more subtle cardiac developmental anomalies that only become apparent later in life. Second, familial associations are rarely obvious. In retrospect, this should be evident from the observations that defined mutations in a single gene can cause seemingly unrelated lesions, compounded by forme fruste or low genetic penetration.
Amongst epidemiological studies, the Baltimore–Washington Infant Study was a prospective surveillance for liveborn cases from 1981 to 1989, with a case-control study to determine aetiological associations. 2,3 More than 4000 cases were identified amongst close to 1 million live births. Some clues about inheritance were obtained, 2–4 but despite suggestive information, the focus of genetic evaluation was on chromosomal anomalies and known hereditable syndromes, and not on identification of specific mutations. Thus, although several teratogenic causes of heart defects have been documented, their underlying causes are unknown. Most recently, prenatal use of angiotensin converting enzyme has been identified as a strong risk factor for congenital defects that include cardiac lesions. 5
Other than obvious associations with chromosomal syndromes, such as atrioventricular septal defects in the setting of Down syndrome, genetic causes have been slow in their discovery and characterisation. Some cardiac conditions have a clear familial component, and include Marfan’s syndrome, Williams syndrome, and Holt-Oram syndrome. 6,7 Recognition of the syndrome produced by deletion of chromosome 22q11 has caused a paradigm shift in how clinicians now think about the genetic contribution to congenital cardiac malformations. 8,9 The deletion syndrome is associated with a host of cardiac lesions, ranging in severity, and mostly involving the ventricular outflow tracts. 7,9 The deletion has been reported in up to three-fifths of those with interrupted aortic arch, one-third of those with common arterial trunk, one-sixth of those with tetralogy of Fallot, and one-tenth of patients with ventricular septal defect. Routine testing is now standard, although its influence on cardiac outcomes is as yet unclear. It is interesting to note that the identification of the most likely causative gene came from studies in the mouse. 10–12
In contrast to clearly defined syndromes, most congenital cardiac malformations rarely occur in families with a sufficient number of affected members to lend themselves to genetic linkage analyses. Also, when familial cases occur, they are often marked by heterogeneity of defect, and affected family members may manifest as cardiomyopathy or arrhythmia rather than congenital malformations. Decreased penetrance and variable expressivity also occur, and suggest that additional environmental and genetic factors may contribute to risk of malformation. Historically, therefore, the risk factors for reoccurrence of the lesions were based on epidemiological studies such as those described above, and were classified broadly into family inheritance and vague environmental considerations. More recently, genetic analyses have determined that even some common types of defects have a genetic component. For example, atrial septal defects, and aortic valves with two leaflets, have been shown to be inheritable. 13,14 The subsequent isolation of the causative genetic mutations substantiated this notion. 15–17
CARDIAC DEVELOPMENT: KEY CONCEPTS
While early anatomical descriptions have provided significant insights into normal cardiac development, modern genetic experimentation with model organisms has been particularly useful in deciphering the anatomical and genetic contortions that the developing heart must undergo to become a formed and functional organ. It has become clear that the genetic pathways that operate in such diverse species as the fruitfly, zebrafish, and mouse are relevant to each other and provide important biological insights that are relevant to human disease. In particular, the fruitfly and the zebrafish have permitted the discovery of previously unknown pathways due to their use in large-scale phenotypic-based screens for discovery of genes. Similarly, genetic manipulation in the laboratory mouse, whose cardiovascular system is nearly identical to that of humans, has allowed profound insight into the mechanisms underlying human disease.
Origins of the Heart
The vertebrate heart arises from paired pools of mesodermal precursors. Cardiac differentiation begins shortly after gastrulation has begun, and the first clear markers of the differentiating heart are apparent near the end of gastrulation, this occurring during the eighth day of development in the mouse. The process demarcates a horseshoe-shaped group of cells called the cardiac crescent (see also Chapter 3 ). These cells will contribute to the atria and left ventricle, while another group of cells more medial within the cardiac crescent will form the so-called second heart field, or lineage, from which the right ventricle, outflow tract, and a portion of the atria will be derived. 18–24 The cells of the cardiac crescent come together at the midline of the embryos, where their fusion and anterior growth leads to the formation of the linear heart tube. This beating structure breaks the symmetry of the embryo, and loops towards the right side as distinct chambers form during the ninth day in the mouse. Looping proceeds during the tenth day, with growth of distinct chambers, giving rise to a heart composed of left and right atria and ventricles. Subsequent steps in cardiac morphogenesis refine the distinctions between each chamber, and separate the left and right sides by growth of septa.
A recent discovery is that the outflow tract, the right ventricle, and a large component of the atria arise not from cardiac crescent–derived myocardium, but from a population of cardiac cells that form more anteriorly—the so-called second heart field. 20–23,25–27 As defined by genetic lineage analysis, the second field originates very early in development from mesoderm expressing the transcription factor Isl1. This lies near the area of the heart-forming mesoderm and is displaced cranially during development of the embryo. A subset of the this area itself, initially called the anterior heart field, is marked by the presence of Fgf10 and Mef2c mRNA. 21,26
This remarkable discovery has fundamentally altered our view of cardiac morphogenesis. Instead of continued growth of a defined population of differentiated cells, uncommitted cardioblasts from the second lineage are actively recruited into the heart, where they differentiate into cardiac cells. In fact, Isl1 -expressing precursor cells have been shown to persist postnatally as cardiac myoblasts. 28 The discovery of the second lineage is also significant from the stance of a disease. To understand lesions involving the outflow tract and right ventricle, we must understand how the factors that regulate their formation from their precursors are coordinated and integrated with the rest of the heart. In DiGeorge syndrome, the defective gene operates primarily in the second lineage, affecting its differentiation and migration. 29,30 Thus, understanding of how the switch in lineage occurs from undifferentiated myoblasts from the second field to differentiated cardiac myocytes has many implications for embryology and disease.
The lineage of the second field has also been shown to be a multi-potent precursor cell, which can give rise to all cardiovascular cell types, including myocytes, endothelial cells, and smooth muscle of the vasculature. 31–33 These multi-potent cells differentiate into the three different cell types presumably in response to local cues, such as growth factors, which instruct a particular gene programme to be activated over another. Additionally, these cardiovascular precursors restrict their potential as they further differentiate. This is a strategy similar to that employed by haematopoietic precursors, which give rise to the different cell types that form blood.
The primary heart field was initially thought to contribute to the entire heart. Although no lineage analysis has been performed, it appears that it is the left ventricle and part of the atria that derive from the primary field. Some evidence for this comes from deletion of Tbx5 , which is largely absent from the second field and its derivatives. 34,35 Loss of Tbx5 in the mouse results in severe hypoplasia of the caudal segment of the heart, the atria and left ventricle, leaving intact the right ventricle and outflow tract. 35 Unlike the complete absence of derivatives of the second field in Isl1 knock-out mice, Tbx5 mutant mice still develop primitive, albeit malformed, structures. Mutations in other genes that affect formation of the heart most severely do not affect early formation of the tube from the first heart field. 23,35–37 The determinants of the so-called primary heart field, and the importance of its differentiation for overall cardiac development, are not known.
Genes That Regulate Formation of the Heart
Beginning with the discovery of the tinman mutant in Drosophila in 1989, several dozen genes have been identified that are critical for various aspects of formation of the heart, from its earliest inception, through major morphogenetic steps, and into postnatal regulation of cardiac function. Most genes encode transcriptional regulators, which turn on or off other genes, or signalling molecules that activate potent intracellular signalling cascades.
Transcription Factors
The fruitfly tinman mutation was identified in flies that did not form any heart at all, the mutation being named after the character in The Wizard of Oz 38 This mutation was in a gene belonging to a family of transcription factors called the homeodomain factors. Shortly after this discovery, vertebrate versions were identified, which were given the less colourful name of Nkx2-5 . It turns out that Nkx2-5 in vertebrates is not essential in itself for formation of the heart, but it does have important functions in early initiation of the cardiac genetic programme, and in formation of the cardiac chambers. 39,40 As will be discussed below, along with many other genes that were initially experimentally defined, NKX2-5 is one of the genes that has been identified as causative in inherited human congenitally malformed hearts. 15 Considerable literature exists on the function of Nkx2-5 , and in many of its functions it interacts with other transcription factors that are important for the normal development of the heart. For example, a factor from another gene family, Gata4 , also plays important roles in heart differentiation, in chamber morphogenesis, and also has additional roles in bringing the two heart fields together. The last role was dramatically evident from its mutation in the mouse, which led to production of a bifid heart. 41,42 And as with NKX2-5 , human genetics has pinpointed GATA4 as a gene that when mutated causes inherited congenital cardiac defects. The primary role of Nkx2-5 in the developing heart is to activate a set of target genes that will execute the correct cellular differentiation program of a variety of types of cells. For example, certain contractile protein genes rely on Nkx2-5 for their initial activation, and the proper development of the conduction system relies on its appropriate function.
Gata4 , as mentioned above, is also a key player in formation of the heart. Indeed, this gene has been shown to be important for such diverse aspects of formation as early differentiation, valvar formation, chamber maturation, and even postnatal function. In fact these same roles have also been ascribed, to varying degrees, to Nkx2-5 . Genes that are active in the early heart usually have binding sites for Gata4 and Nkx2-5 in the regulatory regions, called enhancers , that confer cardiac-specific expression of genes. Gata4 and Nkx2-5 function together to act on these sequences of enhancers, and this interaction provides a degree of robustness and specificity to the system. Many other transcription factors have been defined as important for various aspects of cardiac formation. They often have multiple roles at various times during development, reflecting their potency and versatility.
Growth Factors
Growth factors of many different families are important for several aspects of heart development. In early development, the bone morphogenic factors and the Wnts , two types of developmentally important secreted factors, are key inducers of cardiac development, via their instructive cues that promote expansion of early cardiovascular precursors, and later also the induction of cardiac differentiation from these same precursors. 43–49 The picture is a bit more complicated, as some Wnt signals also dampen cardiogenesis, by slowing the growth of precursors, presumably so that the timing of cardiac differentiation is kept. 43–48,50
Growth factors are also important in later stages of cardiac development, such as valvar formation and septation. 51 Bone morphogenetic proteins are critical for the initiation of the earliest steps in valvar formation, and indeed the dosage of Bmp4 , for example, results in valvar malformations and deficient atrioventricular septation that are reminiscent of human disease. 52,53 Later regulation of valvar morphogenesis relies on a complex interplay between myocardium and endocardium which is regulated by calcineurin-dependent signalling and the repression of vascular endothelial growth factor in myocardium of the valve-forming region. 54
MicroRNA Regulation of Cardiac Development
The transcriptional regulation of cardiac development and its modulatory and instructive signalling pathways are well studied, and their biology is becoming well understood. Less clear is the translational control of cardiac morphogenesis by small noncoding RNAs, such as microRNAs. MicroRNAs are genomically encoded 20- to 22-nucleotide RNAs that function by targeting mRNAs either for translational inhibition or for degradation, leading to an effective reduction in quantity of the protein product. Several hundred human microRNAs have been identified, and some of these have important roles in development that may be eminently relevant to congenitally malformed hearts.
The best characterised example is the microRNA-1 family, comprising miR-1-1 and miR-1-2 . These microRNAs are highly conserved from worms to humans, and are specifically expressed in the progenitor cells of developing cardiac and skeletal muscle as they differentiate. 55 Both are highly expressed in the cells of the outflow tract derived from the second heart field. Interestingly, expression of these microRNAs is directly controlled by well-studied transcriptional regulatory networks that promote muscular differentiation. Cardiac expression is dependent on serum response factor, and expression in skeletal muscle requires the myogenic transcription factors MyoD and Mef2. Consistent with a role in differentiation, overexpression of miR-1 in the developing mouse heart results in a decrease in expansion of ventricular myocytes, with fewer proliferating cardiomyocytes remaining in the cell cycle. Validation of Hand2 as a target for miR-1 suggests that tight regulation of levels of protein may be involved in controlling the balance between cardiomyocyte proliferation and differentiation. MiR-1 and another microRNA, miR-133a , are transcribed in a polycistronic message, therefore sharing common regulation, and both are co-expressed in cardiac and skeletal muscle. As in cardiac muscle, miR-1 promotes the differentiation of skeletal myoblasts in culture, but interestingly, miR-133a has the opposite effect, inhibiting differentiation and promoting the proliferation of myoblasts.
Defects caused by mutations in microRNA genes range from benign to severe. Disruption of the single fly orthologue of miR-1 had catastrophic consequences, resulting in uniform lethality at embryonic or larval stages, with a frequent defect in maintaining cardiac gene expression. 56 In a subset of flies lacking miR-1, a severe defect of cardiac progenitor cell differentiation provided loss-of-function evidence that miR-1 was involved in events leading to muscular differentiation, events, similar to the gain-of-function findings in mice. miR-1 in flies regulates the Notch signalling pathway by directly targeting microRNA of the Notch ligand, Delta, 56 potentially explaining the involvement of miR-1 in deriving differentiated cardiac cells from an equivalency group of progenitor cells. Thus, miR-1 seems to be a muscle-specific microRNA that directs progenitor cells towards a cardiac cell fate by regulating central mediators of determination and differentiation. Although only three miRNAs have been deleted in mice to date, targeted deletion of miR-1-2 resulted in ventricular septal defects, although with incomplete penetrance. 57 In surviving adults, disruption of normal cardiac conduction and cell cycle control was also observed.
Haemodynamics and Formation of the Heart
As the heart forms, it soon begins to beat and pump blood. This occurs at the early stages of cardiac looping, well before chambers have formed and separations between segments of the heart are established. It would seem intuitive that the physical forces of a beating heart would affect its morphological development, but until recently this has been but a concept. In fact, haemodynamic forces are indeed important, and shape not only the normal development of the heart, but produce the secondary defects associated with major structural congenital cardiac malformations.
The initial identification of a role for haemodynamics was in zebrafish, a simple model of cardiac development in which a single atrium connects to a single ventricle. By altering the flow at the inflow or outflow of the zebrafish heart, and imaging the structure and function of the heart, it was determined that altering flow within the heart led to abnormal cardiac looping and defects in formation of the cardiac cushions, indicating that normal intracardiac flow is a key regulator of cardiac morphogenesis. 58 This was confirmed using zebrafish and mouse models in which embryonic contractility is impaired or absent. 59–61 More recent experiments, again in zebrafish, have clearly shown that intrinsic defects in contractility of cardiac myocytes, in combination with external haemodynamic forces, are essential for the normal development of the heart. 62
The most intriguing results on haemodynamics and cardiac development have a direct connection to congenital malformations. It had been observed in a mouse model of laterality defects that include anomalies of the outflow tracts that formation of the arteries in the brachial arches is randomised between the left and right sides. 63 Normally, the arches form initially in bilateral fashion, but the left-sided brachiocephalic artery regresses, leaving only a right-sided artery. It was determined that, in this mouse model, the flow to the brachial arches was randomised to both left and right sides, unlike the usual situation where flow is preferentially directed to the right side. With a combination of surgical and morphological manipulations, it was shown that the altered flow was the likely culprit for the abnormal presence of a left-sided brachiocephalic artery. Thus a genetic defect, in this case involving Pitx2 , not only leads to direct defects in regions of the heart where the mutated gene is expressed, but produces morphological defects secondary to altered flow. This finding has important implications for the understanding of the origin of congenital cardiac malformations.
CHROMOSOMAL DISORDERS
Each body cell contains, in its nucleus, 46 chromosomes. This is its own copy of the blueprint for the body. Of these, 44 chromosomes are common to males and females. These are the 22 pairs of autosomes. The remaining two are the sex chromosomes, X and Y. Aneuploidy is a generic term for any disturbance of the chromosomal complement other than the addition of whole extra sets, which represents polyploidy. Translated literally, aneuploidy means ‘not good set’ and may be defined as the loss or gain of all or part of a chromosome.
Autosomal Aneuploidy
Autosomal aneuploidy, that is, any disturbances of chromosomes other than X or Y, causes major disturbances of embryonic development, with the result that most afflicted fetuses are spontaneously aborted. Among the survivors, the most common form of aneuploidy is trisomy. This is the addition of one whole extra chromosome. Most cases are the result of non-disjunction at meiosis. When the primordial cell divides to produce an egg or sperm, the matching pairs of chromosomes separate so that the gamete contains one of each. This makes 23 in all, and this is known as a haploid set. The fusion of an egg and sperm restores the number to 46. If a pair of chromosomes fails to separate at meiosis, the gamete, and hence the embryo, will have either one too many, or one too few. The latter group abort, as do most of the former, particularly when large chromosomes are involved. Fetuses with trisomy for the smaller chromosomes may reach term, in particular those with trisomy 13, 18, or 21.
Trisomy 21
Of children born with a cardiac defect, 1 in 20 has trisomy 21. 64 It is, therefore, the largest specific aetiological category. Intelligence is rarely in the normal range, the mean quotient being about 50. Such children have an increased incidence of malformation and disease, including duodenal atresia, Hirschsprung’s disease and anal atresia, leucaemia, and defects of the immune system. Those who reach adulthood tend to age prematurely. Of particular interest here is the frequency of cardiac malformations. In a large review of infants with trisomy 21 who had a cardiac defect, just over two-fifths were found to have an atrioventricular septal defect, while three-tenths had multiple anomalies, with persistence of the arterial duct being the second most common lesion, afflicting one-sixth. 65 Review of a necropsy series showed that almost half of infants with Down syndrome had a cardiac malformation, with two-thirds having an atrioventricular septal defect. 66 The strong association of atrioventricular septal defect with trisomy 21 is not seen with other chromosomal anomalies. It prompts the speculation that some important function of growth or adhesion of the endocardial cushions is determined by genes on the chromosome 21, or else abnormal genes disturb the separation of the common muscular atrioventricular junction, since it is the common junction which is the hallmark of the malformation. From a practical viewpoint, the presence of left-axis deviation in a neonate with the features of Down syndrome makes an atrioventricular septal defect very likely.
Mosaic Down Syndrome
About 3% of children with trisomy 21 have a parent who is a mosaic. This means that some of the parental cells have an extra chromosome 21, and this involved one or both gonads. If mosaicism is identified in the parent, the recurrence risk is greater than 10%. 67 It is the existence of such families that accounts for part of the 1% recurrence risk in siblings of a child with trisomy 21. In addition, some parents seem to have an increased risk of non-disjunction, since recurrences of trisomy involving chromosomes other than 21 have been described.
Trisomy 18, or Edward’s Syndrome
Trisomy 18 affects 1 in 3500 newborns. Early death is almost invariable because of the multiple malformations. Survivors are severely developmentally delayed. Typical features are a prominent occiput, low-set malformed ears, and a small jaw, though the appearance is less characteristic than that seen with trisomy 21 or 13. Two additional clinical signs of great value are the unusual clenched fists, and rocker bottom feet. Cardiac defects are present in the majority, most being persistent patency of the arterial duct or ventricular septal defects. A bedside diagnosis is strengthened by examination of dermatoglyphic patterns.
Trisomy 13, or Patau’s Syndrome
One in 7000 newborn infants has Patau’s syndrome. The majority die as neonates. Survival beyond the first year is exceptional. The characteristic clinical features are polydactyly, cleft lip and palate, often bilateral and severe, and hypotelorism associated with malformation of the frontal lobes of the brain, also known as holoprosencephaly. There is a high incidence of cardiac defects, in particular atrial and ventricular septal defects. Abnormalities of cardiac position are relatively frequent, 68 perhaps indicating isomerism of the atrial appendages. As with atrioventricular septal defects and trisomy 21, the high incidence of disturbed cardiac position in trisomy 13 may reflect a specific role for this part of the genome in the determination of laterality.
Aneuploidy of the Sex Chromosomes
The female has two X chromosomes in each cell. The male has one X and the small Y chromosome. The latter determines maleness. The normal development of males shows that only a single copy is required for genes on the X chromosome. Females avoid having an excess by inactivating one of the two X chromosomes in each cell between the 16- and 1000-cell stages of embryonic life, a process called lyonisation . When excess of X chromosomes is present in each cell, all but one are switched off. An embryo, therefore, can tolerate chromosomal complements that contain three, four, or five X chromosomes with relatively little disturbance of development. Apart from the frequent occurrence of persistent patency of the arterial duct in the group with five X chromosomes, cardiac development is not affected. This is not the case when a single sex chromosome is present from the start, as in the 45, X karyotype.
45, X, or Turner’s Syndrome
Though first described by Ullrich, the resultant syndrome is associated with the name of the American physician Turner. Despite being mild relative to most other forms of chromosomal aneuploidy, Turner’s syndrome is associated with particularly heavy prenatal loss. Based on studies of aborted fetuses, it is estimated that the frequency of 1 in 10,000 births represents only 1% of conceptions with the 45, X karyotype. 69 Failure of sexual maturation, and short stature, are associated often with a webbed neck, also known as pterygium colli . Other features are a shark mouth, down-slanting palpebral fissures, and low-set ears. The strong association with aortic coarctation is well known. Aortic stenosis is the most common of a wide variety of other cardiac defects seen rarely in patients with Turner’s syndrome. The prevalence of cardiac defects among 45, X individuals is estimated between 10% and 20%. 70
SYNDROMES INVOLVING MICRODELETIONS: SINGLE GENES OR MORE?
22q11 Microdeletion Syndrome (DiGeorge Syndrome) and Defects of the Outflow Tracts
By far the most important example of a microdeletion is 22q11 deletion. About 3 million base pairs are normally lost in this disorder, resulting in the loss of at least 30 genes. There are segments at either end of the commonly deleted segment that have the same sequence. At meiosis, when the two copies of chromosomes 22 pair, this repeat makes them liable to misalign. If a crossover then occurs, it is possible to produce a chromosome with this segment deleted.
All individuals with this syndrome have facial features, though these may be subtle. Typically those involved will have short and narrow palpebral fissures, which may be up-slanting, a bulbous tip to the nose, which seems pinched because the supporting facial structures are flattened, a small mouth, and small rounded ears. While several acronyms have been utilised to describe this genetic disease, including Shprintzen syndrome, velo-cardio-facial syndrome, conotruncal anomaly face or Takao syndrome, Strong syndrome, and CATCH22, the most descriptive and inclusive name is 22q11 microdeletion syndrome.
A broad range of congenital cardiac malformations are seen in the setting of 22q11 microdeletion syndrome, including tetralogy of Fallot with pulmonary stenosis or atresia, common arterial trunk, and interrupted aortic arch. This range of lesions, in addition to the extracardiac defects found in the syndrome, made it difficult to pinpoint a particular causative gene or group of genes in the minimal 22q11 interval solely based on their known or perceived function. It took clever mouse chromosome engineering studies to pinpoint the likely single gene responsible for the majority of defects in 22q11 microdeletion syndrome. Taking advantage of the similar arrangement of genes between mouse and human, known as synteny, researchers utilised the power of mouse genetics to mimic the 22q11 microdeletion. 71,72 Once this rather spectacular technological feat had been achieved, the same groups refined their analysis to whittle down the interval, in effect doing in mouse what nature had not quite done in humans. These studies, combined with additional mouse models, narrowed down the likely culprit for the majority of defects in 22q11 microdeletion syndrome to a gene for a transcription factor, Tbx1 10,11 Tbx1 encodes a transcription factor that had previously not been functionally characterised, but is a member of the developmentally important T-box gene family. Significant debate surrounded this discovery, as it was considered unlikely that a single gene in the 22q11 minimal interval would result in all the defects in the syndrome, and confounding data from deletions that excluded the minimal interval, and therefore TBX1 , further fueled the debate. The potential for TBX1 as a major causative gene was strongly supported by the identification of TBX1 missense mutations in patients with features of 22q11 microdeletion syndrome, but without a microdeletion. 73 Again, mouse genetics has lent support for the possibility that other genes within the 22q11 critical region probably also contribute to the disease, as deficiency of the 22q11 gene crkl results in similar defect in a mouse model and exacerbates loss of Tbx1 74,75
Although the congenital cardiac malformations seen in the setting of 22q11 microdeletion syndrome were attributed based on inferences to defects in migration of cells from the neural crest migration, Tbx1 is expressed in the second heart field, and is important for its normal expansion. 29,30 The transcription factor Tbx1 appears to affect the neighbouring cells in the neural crest in a non-cell autonomous fashion by promoting secretion of fibroblast growth factor that interacts with its receptor on the cells from the neural crest to affect their proliferation or differentiation.
Williams Syndrome and Supravalvar Aortic Stenosis
The association between supravalvar aortic stenosis and the classic dysmorphic pattern known as Williams syndrome has been established in the literature for many years. 76 It led to speculation that there might be a gene responsible for the cardiac defect that was deleted as part of a microdeletion syndrome. This proved to be correct. Unlike the usual progression from deletion to discovery of the gene, the process was reversed when a patient with supravalvar aortic stenosis caused by a translocation focused attention on the elastin gene carried on chromosome 7. 77,78 Fluorescent in situ hybridisation soon revealed microdeletions in patients with Williams syndrome. Elastin is a major component of the walls of large vessels, and disruption of this gene somehow leads to excessive proliferation of cells in the vascular wall in mice. 79,80 The mechanism by which elastin regulates cellular proliferation is currently unknown. There is continuing controversy about the underlying cause of the characteristic learning disability with the outgoing personality. The chromosomal basis of the recurrent deletion appears to be a set of inversions that promotes recombination. 81
Alagille Syndrome and Peripheral Pulmonary Arterial Stenosis
Peripheral pulmonary arterial stenosis is the hallmark of Alagille syndrome, a multi-system disorder affecting liver, cardiovascular system, spine, eyes, and face. The association of a microdeletion involving chromosome 20p12 narrowed the region of interest and led to identification of the causative gene defect, loss of JAGGED1 82 Mutations in this gene, which encodes a Notch receptor, cause cardiac defects, not only in the context of Alagille syndrome, but also in isolated cases of congenital cardiac disease. 82–84 This gene encodes a ligand for the Notch family of receptors. The Notch pathway is involved in control of a variety of processes, extending from tissue patterning and morphogenesis to cancer. This discovery explains, at a superficial level, the stenotic lesions in the pulmonary vascular tree, along with the other features of the syndrome, such as hypoplasia of the biliary ductules, posterior embryotoxon of the eye, butterfly vertebrae, and the characteristic face. Much work will be needed to understand fully the relationship between the genetic error and the clinical phenotype, but the discovery of the loss of JAGGED1 focuses attention on the correct pathway. Furthermore, the observation that loss of the whole gene produces the same phenotypic spectrum as mutations within the gene that lead to a gene product of reduced size demonstrates that this is a haplo insufficiency syndrome. In other words, the problem arises from loss of half of the gene product.
Defects of Laterality
Defects of laterality are often associated with congenital cardiac lesions, specifically those associated with isomerism of the atrial appendages, or visceral heterotaxy (see Chapter 22 ). The genetic cascades that regulate the situs of internal organs, including the lateralisation of the atrial appendages, have been carefully delineated using experimental models. 85 As defects of laterality in humans can be inherited, it is likely that in many cases single gene mutations are responsible. Indeed, human genetic studies have identified mutations in a few genes that lead to inherited defects of laterality. 85 A search for mutations in these genes in patients with congenital cardiac lesions but in the absence of isomerism that did not have any other feature of laterality defects identified a few rare cases, indicating that they may be a cause of isolated disease. 86,87
Single Gene Defects
In the last decade, human genetic studies have identified several genes that are mutated in those with inherited cardiac disease. All of these are important regulators of cardiac morphogenesis, supporting the concept that the cardiac malformations are primarily a disease of abnormal embryonic organogenesis. These findings have also cemented the notion that most lesions have a genetic origin. In addition, these discoveries have clearly demonstrated that unrelated lesions can be caused by the same genetic defect, resulting in a need to reexamine epidemiological studies from a different perspective. In all cases, the mutations are dominant, as they affect only one of two alleles of the gene. As yet, it is not known how dominant mutations in these genes result in profound aberrations in formation of the heart. They may be related to decreased dosage, or may be due to gain of function effects. It is of paramount importance to understand the molecular consequences of disease-causing mutations, and the mechanism underlying these events, in order to be able to design in rational fashion non-surgical therapeutic interventions for those with congenitally malformed hearts. The future of our understanding of the aetiology of the lesions, therefore, lies in understanding how mutations in these important regulators leads to altered morphogenesis.
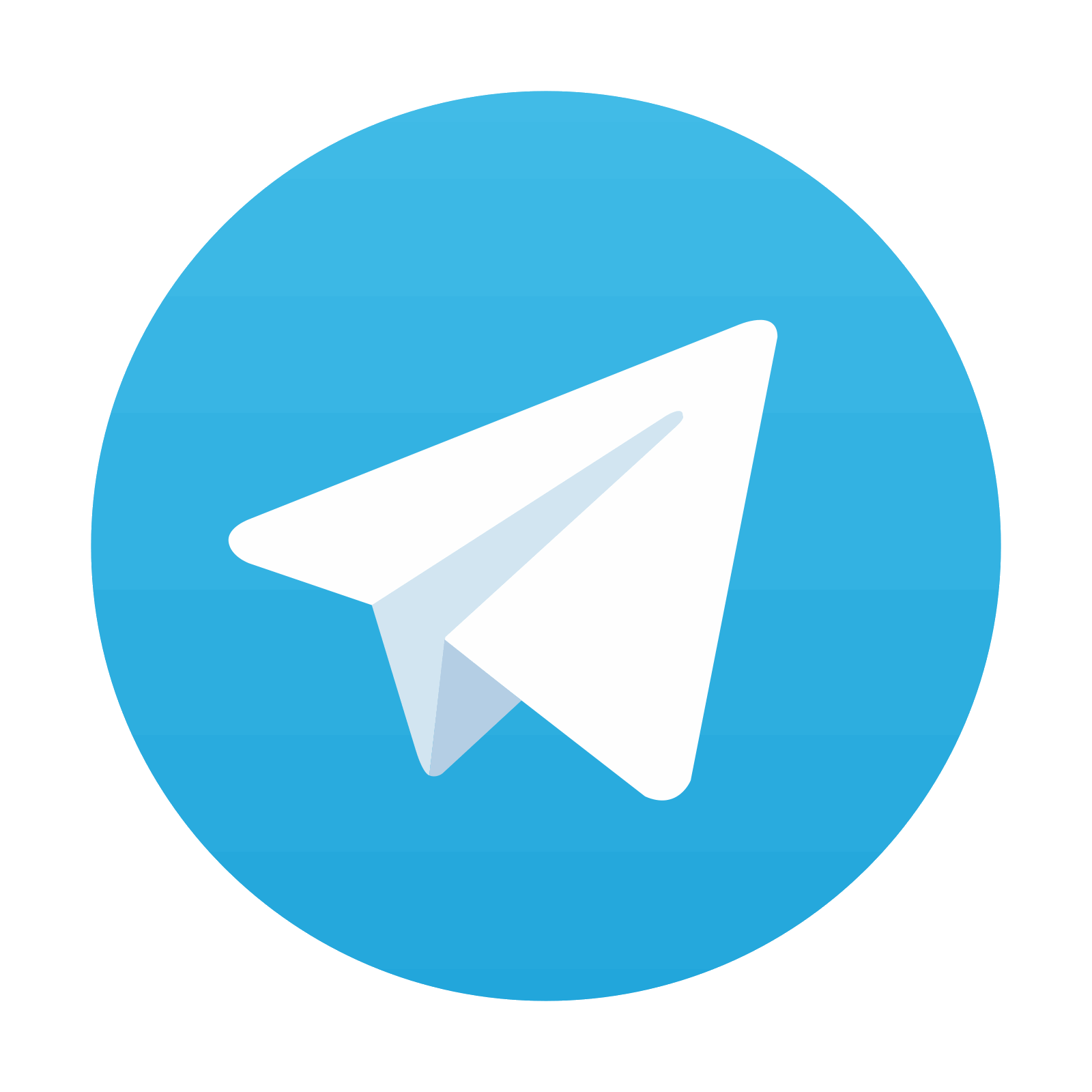
Stay updated, free articles. Join our Telegram channel
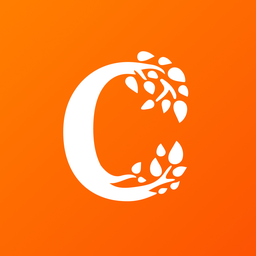
Full access? Get Clinical Tree
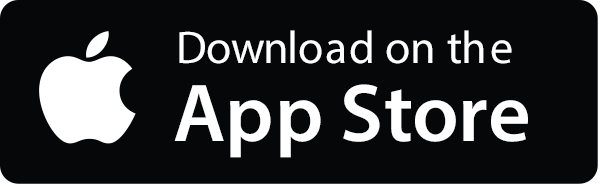
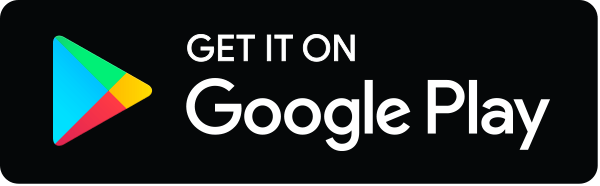