Fig. 4.1
Representative intraventricular blood flow profiles of the right (RV) and left ventricle (LV) at diastole and systole in a healthy control. The main trajectories of blood flow are indicated by the arrows and there are important differences between the RV and LV. The filling of the LV resembles the filling of a wine glass, where during RV filling the majority of blood is directed towards the outflow tract. Filling patterns and associated loss of kinetic energy are substantial in disease (see other figures)
In RV disease, one of the most frequent clinical applications of VEC MRI is the assessment of RV volume or pressure overload due to valve dysfunction (regurgitant fraction), pressure gradients, or shunt volumes. The current gold standard is to measure blood flow through one single 2D plane; this can be measured using 1.5 and 3.0 T scanners [8, 9]. 2D VEC CMR was shown in various studies to be a robust tool to quantify blood flow velocity profiles and derived volumes yielding low inter- and intraobserver as well as low inter-study variability [2, 8, 10]. Flow measurements can be performed during a breathold or even in real time [11, 12].
However, the accuracy of VEC MRI has several technical limitations. These include, amongst others, spin dephasing in chaotic turbulent flow [13, 14]. The method’s reliability can be impacted in vessel segments with local chaotic flow, for example, due to vessel narrowing [15, 16]. A limited spatial resolution can restrict its use in small moving vessels like the coronary arteries. In addition, the assessment of pressure gradients using the simplified Bernoulli equation can induce errors, because this equation can only be applied, like in Doppler echocardiography, to nonviscous fluids. Only the convective and transient effects can be considered but not the viscous loss and turbulent ones. Because the pressure drop in stenotic vessel segments is mainly affected by the momentum loss due to vortex formation behind a stenosis, it is well resolved by the Pressure-Poisson equation.
Recently, four-dimensional flow applications (4D VEC CMRI) that measure flow velocities in a three-dimensional volume were introduced and validated [17, 18]. The validity of 4D compared to 2D VEC CMR for measuring quantitative flow was shown [19, 20]. The theoretical advantages of 4D versus 2D VEC CMR are manifold because flow analysis in the areas of interest is done during postprocessing. Therefore, time consuming and sometimes error prone selection of 2D image planes is omitted [19, 21]. In addition, velocity profiles are measured in all three dimensions which enables us to derive a multitude of new parameters like relative pressure-maps or wall-shear stress {Markl, 2010 #3} [22–24].
In addition, the analysis of 4D blood flow opens new doors for the noninvasive assessment of pressure fields (maps) [17, 25]. This method provides time-resolved blood flow velocities in a three-dimensional volume that can cover the entire heart and great arteries. From these velocity fields, dynamic pressure differences can be computed by solving the Pressure-Poisson equation [25].
Lt-Gd-Enhancement, T1-Mapping, and T1/T2 for the Study of Fibrotic Tissue, Inflammation, or Fat Infiltrates
The late gadolinium enhancement (LGE) approach is based on impaired washout of extracellular contrast agents from areas with focal myocardial fibrosis. This method has been originally developed for visualization of scars in ischemic heart diseases; however, it has been extended to many other applications. In the pressure overloaded RV, LGE is observed in ventricular insertion points, particularly in advanced cases of pulmonary hypertension [26]. The extent of hyperenhancement is related to the right ventricular systolic dysfunction [27, 28]. In PHT, the extent of delayed enhancement of myocardial mass is related to right ventricular dysfunction correlated with worse right ventricular function and hemodynamics. Other studies also found hyperenhancement in patients with Tetralogy of Fallot, however, without clear clinical correlation [29, 30]. However, LGE does not allow quantitative assessment and misses diffuse fibrotic processes. Therefore, alternative methods like T1-mapping were developed that use parametric strategies to also detect and quantify diffuse fibrosis [31–34] (Fig. 4.2). The assessment of RV fibrosis may provide prognostic information, however this remains to be studied in great detail.


Fig. 4.2
Diffuse fibrosis of the myocardium as visualized and quantified by T1-mapping techniques
T2 or combined T2/T1 weighted imaging (STIR) are sensitive to water and therefore allow the identification of increased water content, of myocardial edema, for example, in the setting of myocarditis [35]. The extent of edema can correlate with the clinical state and can be of prognostic value [36]. In addition, these techniques are of value for the study of cardiomyopathies [37]. T1 or T2 weighted images in combination with fat-suppression techniques are also part of the CMR criteria which characterize RV dysplasia [38].
CMR Feature Tracking, Tissue Maps, and Tagging for Measuring Tissue Deformation and Regional Wall Motion
In analogy to tissues Doppler echocardiography, CMR techniques allow us to measure the amount and/or velocities of myocardial tissue deformation (strain and strain rate, respectively). Their derivate are considered parameters of myocardial performance and diastolic relaxation. Compared to tissue Doppler, all CMR methods are limited by low temporal resolution but have the large advantage of operator independency and comprehensive 3D assessment. By CMR, tissue deformation and regional wall motion can be measured by the following techniques: Feature tracking is a postprocessing method, comparable to Doppler spackle tracking, that can be performed using conventional cine MR images as they are acquired during most CMR routine studies [39–41]. This method is appealing because it does not require additional scanning. Other methods, require extra dedicated scanning, are CMR Tagging or phase-contrast based tissue maps [42–45]. 3D Tagging techniques are independent of morphology and operator [46]. Newer techniques have been developed to allow single heartbeat assessment of RV strain [47].
MR Spectroscopy: A Research Tool for Imaging Cell Metabolism
Impaired high-energy phosphate metabolism may play a critical role in the pathogenesis of RV failure due to PAH. However, due to technical and sensitivity reasons, the use of 31P-MR spectroscopy to characterize the energy metabolism of the right ventricle in the human heart does not yet play a significant role [48]. Higher field strength and improved techniques may in the future allow MR spectroscopy to play a role in the assessment of patients and their response to therapies. With the latest advancements in 3 T and even 7 T MR technology, multi-voxel spectroscopic (chemical shift) imaging of phosphorous metabolites for the right ventricle comes into reach. This would allow us to investigate cellular energy metabolism and clarify some of the pathophysiologic mechanisms which underlie the different phenotypes of right ventricular dysfunction and failure. The evaluation of metabolic pathways is also the subject of the nuclear medicine techniques described in Chap. 13.
Angiography, 3DWH, and Diffusion-Tensor Imaging for the Assessment of Anatomy at a Macro-and Microscopic Scale
MR angiography and 3D whole heart (3DWH) imaging are widely used in the clinical routine to define the anatomy of the RV and the thoracic vessels. The latter technique does not require contrast media. This is of interest because in patients with renal failure gadolinium based contrast media can induce nephrogenic systemic fibrosis, which appears to occur with a lower incidence in children [49]. MR angiography is a fast and precise method for visualizing the pulmonary vasculature. 3DWH is more time consuming but provides also information about the intracardiac anatomy, including the proximal coronary artery; this is particularly important when planning percutaneous pulmonary valve replacement. 3DWH also offers the opportunity to visualize a complex anatomy in the form of virtual or printed cast models that can be of value for the planning of surgical treatment (Fig. 4.3) [50].


Fig. 4.3
Three-dimensional reconstruction of intraventricular anatomy based on 3DWH MR imaging method. Shown is a Swiss-cheese VSD seen from the left ventricle
Diffusion-tensor imaging allows us to visualize and quantify the 3D architecture of myocyte chains (fibers) (Fig. 4.4). This non-destructive method offers unique opportunities to study the biomechanical properties of the ventricles [51]. Recent studies showed that the RV lacks the extensive zone of circular myocytes seen in the mid-portion of the left ventricular walls. Without such structural support, the right ventricle is ill equipped to sustain a permanent increase in afterload [52]. Because of its long image acquisition time, DTI cannot be applied in the beating heart. Still, this method is valuable for studying the pathophysiology of the RV.


Fig. 4.4
Diffuse tensor MR images (DTI) of an ex vivo murine heart (top-down view). The aggregated myocytes (“fibers”) are visualized for the left and right ventricle. The right panels show the course of a bundle of selected aggregates through the myocardium (seen from top-down). DTI is not suited for in vivo imaging but it provides valuable information that are essential to understand the biomechanical adaptation of the RV to overload conditions. This information can be then used and integrated into electro-biomechanical models of the heart allowing simulation of pathophysiologically important conditions (right panel; Courtesy Gernot Planck, University of Graz, Austria)
Image Based Modeling
Image based modeling methods have an enormous potential. Pushed to their present limits they can be useful when it comes to testing patient-specific treatment strategies, simulating the evolution of the disease and predicting the outcome of catheter intervention, surgery, or the response to personalized pharmacological treatment [53]. For these reasons, modeling methods have been developed in the past for multi-biological scales. Biomedical models focus on the simulation of processes at the omics level, the level of cell physiology or tissue metabolism. All these models are still subject to basic research. In contrast, Imaging based models have already reached a level of maturity and advanced them from a purely experimental stage of research to first clinical applications.
In this context, CMR provides the high quality quantitative information of anatomy and function that are required for accurate and robust modeling. These models allow to simulate myocardial deformation (using electro-biomechanical principles, Fig. 4.4) [54, 55], blood flow (by applying computational fluid dynamics, Figs. 4.5 and 4.6) [56–58], or ventricular pressures and volumes (by applying lumped-parameter models) [59, 60]. One of the current important innovations is the application of computational fluid dynamics in congenital heart disease. First human studies showed their great potential to simulate and predict energy loss of blood flow in the Fontan circulation (see Chap. 8) or the pressure drop across the stenosis in aortic coarctation before and after intervention (Fig. 4.5) [60–62]. The same principles of simulation before treatment planning are applied in current research to the pulmonary artery system (Fig. 4.6). Thus, image based modeling opens new avenues where imaging transcends a purely diagnostic method and becomes a tool to simulate disease pathways, plan patient-specific treatment strategies and predict their hemodynamic outcome. Future research will have to validate these methods, and prove that the use of these methods and tools improves outcome.



Fig. 4.5
The figure shows the principles of blood flow simulations in a patient with aortic coarctation. Large panels: computational fluid dynamic based simulation of blood profiles before, at virtual stent placement, and after real treatment. Small panels: anatomy and the position of virtual stents (red color). Such simulation allows to test the best type of treatment that results in optimum flow profiles

Fig. 4.6
Image and flow based modeling of pulmonary blood flow and wall shear stress of virtual stenting and pulmonary valve replacement. The simulation indicated that the existing pressure gradient could be slightly decreased, however, due to the geometric constellation without improving blood flow volumes through the right pulmonary artery. The predicted values were confirmed after treatment
Differential Analysis of RV Form and Function by CMR
RV Systolic Pump Function at Rest and During Stress
Despite enormous progress in quantitative echocardiography CMR is still considered the gold standard for the assessment of RV systolic pump function. Low inter-study variability makes CMR reliable for follow-up studies [1, 2, 63–65]; however, CMR is not the preferred tool for serial assessment in short intervals due to limitations in costs and availability.
Cine CMR and VEC CMR based pediatric normative percentile values of biventricular and biatrial pump function became recently available [66–68]. Several studies have defined thresholds for RV dilation due to valve insufficiency in Tetralogy of Fallot. New research highlights gender differences in the response of the RV to pressure and volume overload in children and adolescents [69, 70]. In addition, there is growing evidence that the RV should not be assessed in isolation but a functional assessment should include its subcompartments (inflow, trabecular-apical, and outflow tract). This is of great clinical importance because the different components contribute differently to RV remodeling and dysfunction [30, 51].
Systolic pump function analysis of the RV and LV is conventionally performed based on short-axis cine CMR views. More recent reports propose a strict transverse (axial) imaging plane, particularly when the study is focused on the RV [2, 71, 72]. If standardized protocols are used for image acquisition, the inter-study variability was reported to be low, no matter whether images were acquired in short-axis or transverse planes [2, 51, 64, 65, 73–75]. In contrast, intraobserver and interobserver variability are an important source of error that must be considered for the comparison with follow-up studies [1, 2, 73].
Systolic pump function and their reference values are based on measurements at rest. Recent reports, however, point out that the functional reserve (ability to increase stroke volumes under stress) can be a marker of disease progression [76]. In CMR, assessment of the functional reserve can be done by including a pharmacological stress with dobutamine [77, 78].
RV Diastolic Function
The role of LV diastolic dysfunction in heart failure has been investigated and is now appreciated. However, there is about a paucity of data regarding the contribution of RV diastolic dysfunction to failure. Several studies have observed RV stiffening in the pressure overloaded RV [79–81], but in contrast to the straight forward analysis of systolic pump function, the assessment of diastolic function requires a much more sophisticated approach. Time–volume relations of the cardiac chambers that can be obtained from cine MRI or blood flow measurements are suitable to study the interplay between atrial, ventricular, and pulmonary function during ventricular filling [79, 80]. In addition, after surgery, pericardial scars seem to importantly impact RV filling [82].
The analysis of time–volume curves is cumbersome and not recommended for clinical use. Intrinsic parameters of myocardial relaxation can be determined by end-diastolic pressure–volume relations using the same CMR approaches as described in the section below. In addition, the assessment of diastolic strain-rates by CMR feature tracking, tissue phase mapping, or tagging is feasible. However, when it comes to diastolic function analysis these methods are limited due to the relatively low temporal resolution of CMR compared to tissue Doppler techniques.
A surrogate of diastolic dysfunction may be the extent of myocardial fibrosis. Novel T1-mapping techniques can quantify the degree of diffuse fibrosis. This method is also described in more detail below.
RV Performance Beyond Pump Function
Tissue deformation: Myocardial strain and strain rates can be measured by cine CMR based feature tracking, myocardial Tagging, or tissue phase maps [39–46]. In experimental models and in patients with RV pressure overload due to PAH, global and regional RV strain is reduced already during the early adaptive period, presumably due to hypertrophy of the RV wall [44, 47].
Pressure–volume relations: The gold standard measurements which describe ventricular function and myocardial performance are the end-systolic and end-diastolic pressure–volume relations. For research purposes such relations can be determined by conductance or impedance catheters which measure ventricular volumes and pressures simultaneously {Baan, 1992 #38} [83]. However, the accuracy of the RV studies with these catheters is limited because they require a symmetrically shaped ventricular cavity studies for volume measurements. As an alternative, CMR guided catheterization makes use of invasive pressure measurements and combined with cine CMR derived ventricular volumes [84, 85]. From such data end-systolic pressure–volume relationships can be computed and myocardial contractility as well as diastolic compliance can be obtained. Different CMR methods have been introduced and validated; they either alter ventricular loading or are based on estimations from a single steady-state beat [12, 84–87]. Other concepts, that may facilitate clinical use, are fully noninvasive approximations [88, 89] or combined pressure–volume measurements by real-time 3D echocardiography [90].
Kinetic energy: As stated above, pressure–volume relations are well established and assess RV work load and the efficiency of the mechanical work. However, this approach neglects important energy losses when the ventricle pumps blood into the arterial system. During diastolic filling blood enters the ventricular chambers with a given amount of kinetic energy. In the healthy heart, the intraventricular blood profiles show characteristic patterns that allow the blood to keep its momentum during systolic ejection (Fig. 4.7). In this case, the energy loss due to uncontrolled vortex formation is minimal. However, in the presence of valve disease local turbulence occurs which changes the characteristic intraventricular blood flow patterns. Moreover, in the dilated ventricle, vortex formations cause a significant loss of the kinetic energy which makes up an important part of the total energy consumed by the blood pumping heart (Fig. 4.7) [91–93]. The energetic efficiency is therefore directly determined by pressure load, chamber size, and blood flow kinetics. This should be kept in mind when designing a treatment plan in order to prevent the transition from compensated RV dysfunction to frank failure.


Fig. 4.7
The graphs show representative RV time–volume curve (time normalized), blood flow kinetic energy (KE) curves (in mJ) of a healthy control, and a patient with Tetralogy of Fallot. The patient had dilated RV due to pulmonary regurgitation (PR). There is substantial increase of energy loss in the diseased RV. The left panels show color coded 4D flow velocities during mid-diastole and early systole measured by velocity-encoded MRI. High velocities (red color) represent high kinetic energy of the flowing blood. The images illustrate nicely that in the patient there are high kinetic energies due to pulmonary insufficiency. In addition, the mobilized kinetic energy at systole is much higher than in the control
RV Tissue Characterization (Including Focal and Diffuse Fibrosis) and Perfusion
CMR is regarded as the reference method for noninvasive evaluation of myocardial tissue properties. Given the current MR technology, in-plane resolution ranges from 1.0 × 1.0 to 2.0 × 2.0 mm2. Reliable tissue analysis requires a structure width of at least three pixels, translating into a minimal wall thickness of 3–6 mm depending on the MR technique to be used. While these conditions are not always met in normal right ventricles or dilated right ventricular disease, such as ARVD (see Chap. 16), right ventricular hypertrophy caused by pressure overload leads to wall diameters that are in the majority of patients sufficient for tissue analysis.
One of the most intriguing aspects of CMR tissue analysis in the context of the pressure overload (pulmonary stenosis or PAH) is its ability to detect myocardial fibrosis. The late gadolinium enhancement (LGE) approach allows qualitative visualization of scars but is missing diffuse myocardial fibrosis. Extensive animal and human research is ongoing to develop and apply T1-mapping methods to study the presence and functional impact of diffuse fibrosis on the ventricular function [32–34].
Adenosine stress perfusion MRI has been successfully used to quantify myocardial perfusion reserve (MPR) which is a significantly reduced in sclerodermia patients with PAH as compared to scleroderma patients without PAH [94].
Pulmonary Vascular Function
Improved knowledge of pulmonary vascular function is an essential part of the work-up of RV function. Quantitative blood flow volumes can be obtained using 2D or 4D VEC MRI (see above). These flow volume data provide quantitative information about pulmonary perfusion, left–right lung perfusion mismatch, intracardiac shunt volumes or collateral flow through aorto-pulmonary or veno-venous collaterals [21, 95–98]. Perfusion and shunt volumes can be determined for baseline conditions. In addition, their response to pharmacologically induced changes, such as selective pulmonary vascular dilators, can be determined [99, 100].
The pulmonary arteries in PAH are characterized by typical morphological changes and the size and growth of the pulmonary arteries can be determined by cine CMR or MR angiography. In patients with Fontan circulation, impaired growth of the pulmonary arteries despite normal somatic growth has been demonstrated [101].
The combination of pulmonary flow volumes combined with invasive pressure data permits us to calculate the pulmonary vascular resistance. While thermodilution based measurements are known to have limited reproducibility in the presence of RV dysfunction, oxymetry is time consuming and invasive as repeated catheter manipulations are required. In addition, the presence of shunt and collateral flow provide only estimate data. As an alternative, MRI guided catheterization was introduced and validated for simultaneous assessment of VEC MRI flow and invasive pressures [99, 100]. Current directions go to combine MRI flow with sequentially obtained pressures from right heart catheterization [102]. The time window should be small and physiologic condition (e.g. volume loading) similar between the two studies. An estimate of pulmonary resistance may also be noninvasively derived from MRI flow measurements [103].
The distensibility of given pulmonary vascular segments is the difference in the vessel cross-sectional area during diastole and systole and can be measured by Echocardiography or cine MRI. Combining distensibility with flow derived parameters allows the calculation of vascular compliance or stiffness, a parameter that has been shown to predict mortality in PAH [104]. This approach uses pulse wave velocities that are either based on the transit time of flow or the flow area [105]. The assessment of total pulmonary vascular compliance is a valuable and more global index of pulmonary vascular function that requires assessment of high fidelity invasive pulse pressures [106].
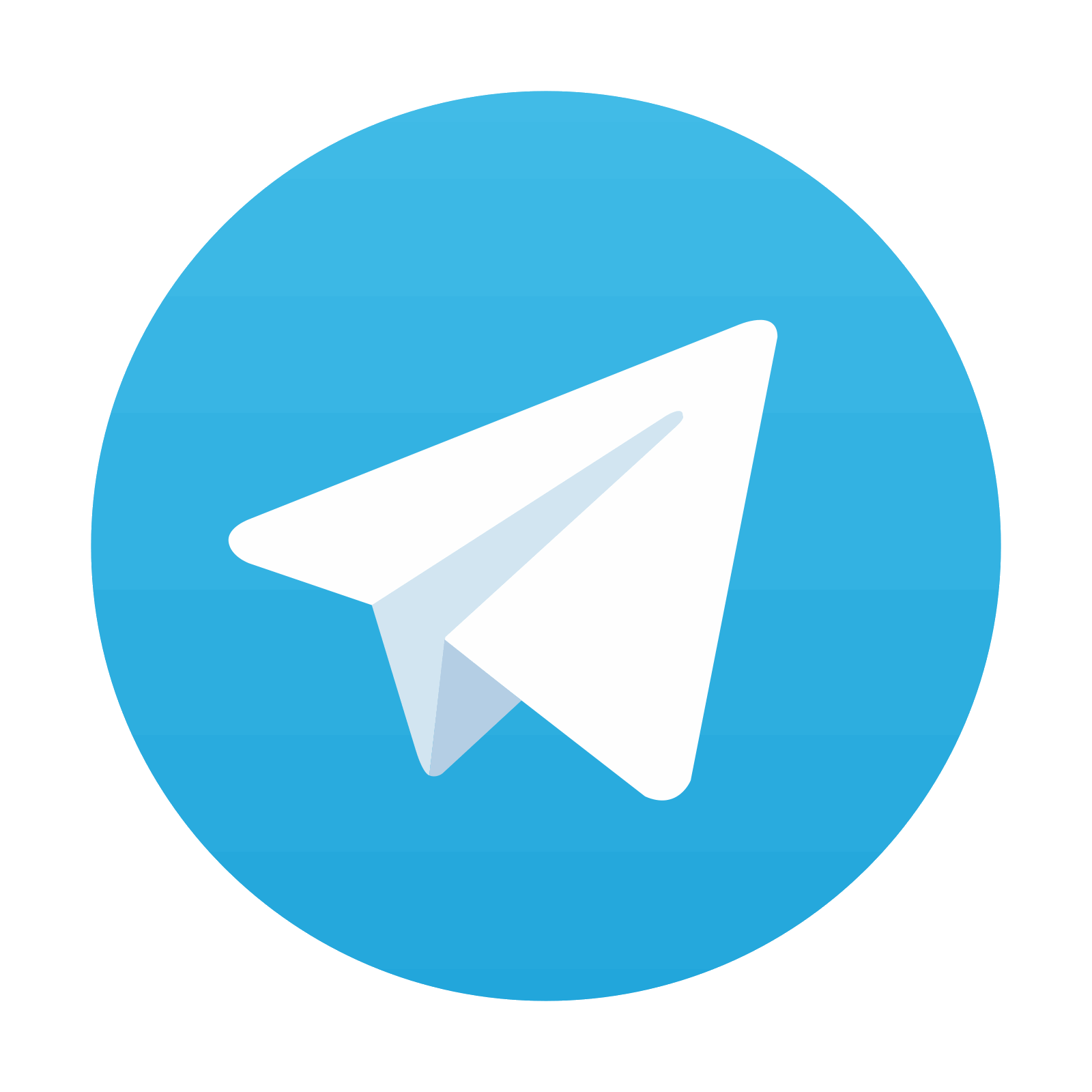
Stay updated, free articles. Join our Telegram channel
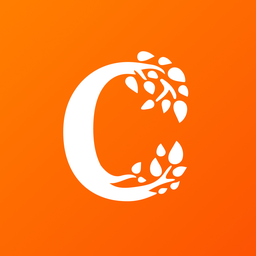
Full access? Get Clinical Tree
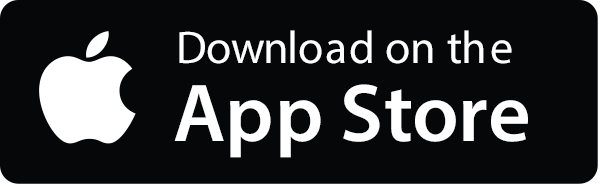
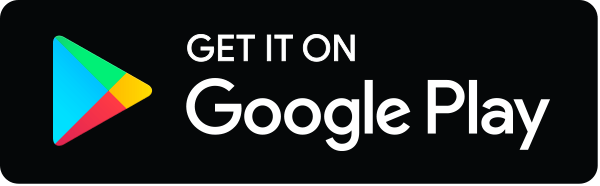