Fig. 22.1
Examples of parametric mapping in various diseases. (a) Acute myocardial infarction imaged at 3 T: Late enhancement with transmural myocardial infarction. T2– and native T1 mapping with elevated T2 and T1 relaxation times in the infarcted area, whereas postcontrast T1 relaxation time is reduced there. (b) Takotsubo cardiomyopathy obtained at 1.5 T: Cine imaging shows apical ballooning. In the apex, T2-weighted imaging shows increased signal intensity, and on T2 mapping a regional elevation of T2 relaxation times, while there is no corresponding necrosis in the late enhancement images. (c) Anderson Fabry Disease (3 T): Cine imaging shows inferolateral hypertrophy. Late enhancement detects intramural signal increase. T2-weighted imaging defines myocardial edema there, also represented by the elevated T2-relaxation time on T2 mapping. On native T1 mapping, T1 relaxation time in the myocardium (excluding the late enhancement region) is significantly reduced compared to normal (984 ms vs. 1,157 ms [9]), which is typical for Fabry disease [11]. In the area of late enhancement, native T1 relaxation time is elevated. (d) Myocarditis (1.5 T): Late enhancement imaging shows the typical subepicardial inferolateral signal increase. On T2-weighted imaging, regional edema is detectable. T2– and native T1-mapping show elevated T2 and T1 relaxation times there, while postcontrast T1 relaxation time is reduced
T2-mapping is based on the acquisition of a set of single-shot images with different echo times or a set of images with different T2 preparation times. The arrangement of the signal intensities of each image pixel over the different echo times or preparation times again facilitates the pixelwise quantification of T2 from an exponential fit, which is then summarized in one T2-map [16]. Mean myocardial T2-relaxation time at 1.5 T is about 55 ms [17] and at 3 T about 45 ms [9], but differences between sequences and scanners have to be considered. In contrast to T1, T2 theoretically does not change over field strengths, however stronger magnetic susceptibilities at higher field strengths lead to an increased signal loss over time by diffusion effects. T2 relaxation times have been observed to increase in acutely injured and inflammatory myocardium like in acute myocardial infarction, myocarditis and Takotsubo cardiomyopathy [18, 19]. Thereby, T2 relaxation times are about 12 ms longer in the involved myocardium compared to remote segments due to the increased free water content (Fig. 22.1).
As an outlook, the term “magnetic resonance fingerprinting” has recently been introduced to measure various tissue parameters of the brain within one MR acquisition [20]. If this concept can be translated to the myocardium, it has the potential to complement parametric mapping of the myocardium. This technique promises to also measure peripheral parameters, such as magnet field and transmission field non-uniformities, which can be pronounced at higher magnetic field strengths.
Cardiac Diffusion MRI
The assessment and visualization of tissue microstructure has moved into the focus of magnetic resonance research during the past years. Diffusion MRI provides non-invasive means to extract structural tissue information and can be used to depict tissue fiber architecture [21, 22]. This technique uses strong, hardware demanding motion encoding magnetic field gradients. Molecular motion along these gradients leads to a pronounced dephasing of the magnetization and thus a loss of signal. Diffusion MRI exploits the fact that water molecules predominantly move along tissue fibers and nerves, which allows their visualization. Diffusion tensor imaging (DTI [23]), q-ball imaging [24], and diffusion spectrum imaging (DSI [25]) are techniques based on diffusion weighted MRI that aim at the characterization of tissue by assessing fractional tissue diffusion anisotropy, mean diffusivity, and by determining the angular alignment of the tissue fibers. Conventionally, at least two different diffusion weightings are required together with a set of different diffusion directions to provide angular resolution.
MR tractography is the visualization of the tissue fibers in a tractogram. This technique uses three-dimensional modelling of data collected by diffusion MRI and is already well established for neural fiber tracking in the brain. Histological studies have shown that myofiber tracts at the endocardium form a right handed helical structure (positive angle), mid-myocardial fibers are circumferential (zero angle), and epicardial myofibers show a left-handed helical structure (negative angle) [26, 27]. These fiber tracts form laminar sheets that allow the heart muscle to contract. Figure 22.2 shows the fiber architecture of the left ventricle of a human adult heart ex-vivo [28]. Initial in-vivo results have already been presented in early studies using data retrieved from two-dimensional acquisitions [29]. The quantification of microscopic molecular diffusion in-vivo by diffusion MRI in the heart is however challenged by its superposition of cardiac and respiratory motion as well as by signal-to-noise constraints. With the improvement in MR hardware and imaging techniques, human whole-heart in-vivo diffusion quantification and tractography are on their way [30].
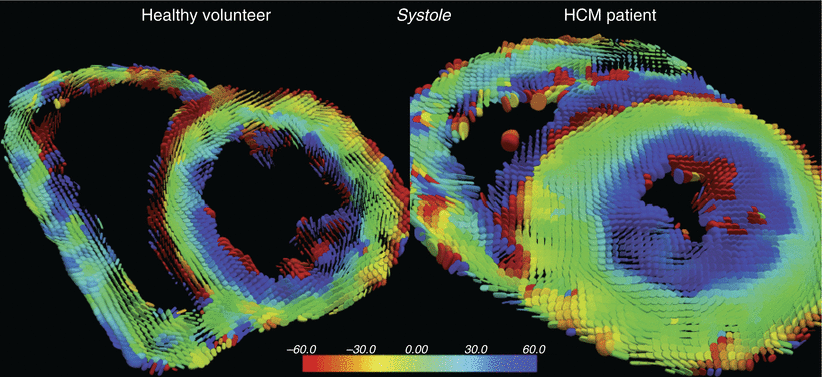
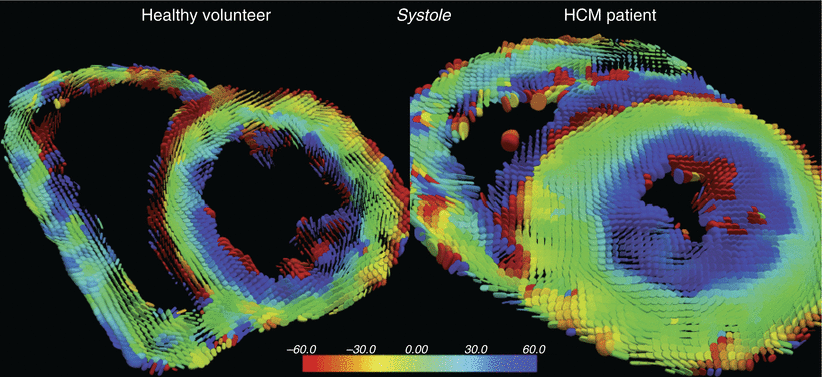
Fig. 22.2
In vivo cardiac diffusion tensor imaging of a patient with hypertrophic cardiomyopathy and a healthy volunteer. Superquadric glyph maps color coded helix angle (Image courtesy of the Cardiovascular Biomedical Research Unit at the Royal Brompton Hospital, Imperial College of London, UK)
Cardiac diffusion MRI techniques are promising for the assessment of cardiac diseases that exhibit structural damage and remodeling of the myofibers, including myocardial infarction and hypertrophic cardiomyopathy. There is evidence that the heart muscle structure on a cellular level is linked to whole-organ functionality [31, 32] and provides essential biological information.
Myocardial Fat Detection
Detection of myocardial fat was one of the first applications in myocardial tissue differentiation applying spin echo sequences with and without fat suppression. It is a basic tool in intra- and para-cardiac mass assessment by differentiation fat and water. The simplest example is the distinction of lipoma and cysts. Besides basic applications, clinicians expect significant diagnostic advances based on the detection of fatty infiltration in the right ventricle, e.g. in arrythmogenic right ventricular cardiomyopathy (ARVC). This disease has a certain risk profile especially in young patients and athletes; so far an accurate noninvasive differentiation is lacking. But, most cardiac injuries including ARVC have different compounds including but not limited to fat. In ARVC, fibro-fatty infiltration is a key finding seen in autopsy and is considered crucial for diagnosis [33]. However, conventional CMR-techniques only allow fat or fibrosis detection by applying non-contrast-enhanced or contrast-enhanced techniques. Diagnosis is challenged by the fact that both pathologies appear bright in widely used late gadolinium enhancement (LGE) techniques.
Recently published techniques offer the opportunity to overcome this limitation. Kellman et al. described a multi-echo dixon fat and water separation method for cardiac application [34], which were discussed in detail recently [35]. Nezafat et al. presented a validation from phantom experiments to initial patient studies applying fat-water separating techniques [36].
First clinical applications have shown promising results. Several groups have described the presence of LGE in dilated cardiomyopathy as a prognostic marker related to clinical outcomes. Lu et al. were able to detect fat deposition within scar tissue and showed a relation to a worse remodeling of the left ventricle in this cohort. Patients with fat deposition showed higher ventricular volumes and a larger scar size [37]. The described sequence was also applied in patients with different risks for atrial fibrillation following the ARIC risk score. The ARIC risk score was developed in a trial evaluating the Atherosclerosis Risk in Communities. Atrial fibrillation is a common phenomenon in the aging society and the different therapeutic options are not well stratified and are suffering from different challenges. One missing piece is the non-invasive characterization of the atrial wall, as the atrial wall can also consist of fat and/or fibrosis compounds. Tereshchenko et al. have shown that the fatty substrate in the atrial walls including the septal wall has an association with the ARIC risk score [38].
Besides the dedicated technique, the interest in fat detection is also reflected by a recently published postprocessing algorithm, which is based on standard cardiac CINE imaging. The bright fat-signal in the pre-contrast cine does have certain clinical value, but does not allow for the differentiation of fat and fibrosis [39].
Fatty alterations of the myocardial tissue are not only known in cardiomyopathies, but also in coronary artery disease mainly in chronic myocardial infarction, and in valvular disease. That knowledge is based on basic work in pathology, whereas experiences in noninvasive detection by CMR are limited. The fast and reliable detection and differentiation of fat and fibrosis is expected to be a major step to enhanced risk stratification.
It should be mentioned that cardiac spectroscopy to quantify myocardial triglycerides (MTG) by magnetic resonance proton spectroscopy (MRS) seems to open a new area in risk stratification of the healthy population. Conservative risk factors for coronary artery disease are well-known and widely established, but are often not sufficient. Several groups already published MTG-MRS results at 1.5 and 3 T. As expected, MRS has a higher SNR at 3 T, potentially leading to higher accuracy [40]. Several technical challenges affect the application of MTG-MRS including navigator-gating [41]. Despite the fact that the MTG-distribution is inhomogeneous throughout the myocardium as shown ex-vivo [42], several groups have shown the impact of different conditions on diagnosis. MTG is not only a predictor for the development of diastolic dysfunction in different known risk diseases like diabetes mellitus [43], but also in the healthy aging population as shown for men [44]. In obese female a correlation to the cardiorespiratory fitness was shown [45] and the influence of moderate dietary induced weight loss was detectable [46]. Nevertheless, MTG-MRS needs significant technical improvement in terms of reliability and reproducibility for application in daily clinical routine.
Myocardial Mechanics Analysis
Almost all cardiac diseases have an impact on cardiac function. Assessing systolic myocardial function is mainly based on quantifying ventricular ejection fraction and visually determining the presence of wall motion abnormalities. These parameters are often abnormal only late in the course of the disease and they tend to overlook regional abnormalities. Diastolic dysfunction accounts for a high percentage of patients with heart failure, but its assessment is a challenge. Therefore, attempts are being made to evaluate systolic and diastolic motion of the myocardium in detail using various new approaches. These efforts include myocardial tagging [47, 48], phase-contrast velocity encoding [49], displacement encoding (DENSE [50]), strain encoding (SENC [51]), and a combinations of these techniques.
Tissue Phase Mapping
Tissue phase mapping measures the velocity of the myocardial motion using time-resolved three-directional phase contrast imaging. Longitudinal, circumferential and radial velocities can be obtained [52]. Image acquisition is mainly done with respiratory navigator and ECG-gating, with a temporal resolution as high as possible. Postprocessing requires segmentation of myocardial segments, finally enabling the calculation of peak systolic and diastolic velocities, time-to-peak periods, as well as composite parameters like myocardial twist. Differences between men and women, old and young, as well as normotensive and hypertensive patients have been reported [53, 54].
Feature Tracking
Feature tracking is comparable to speckle tracking used in echocardiography. Specific software loads conventional steady state free precession cine images to detect features, such as the apparent cavity boundary or tissue patterns related to the endocardial contour. The software tracks these features from image to image and thus quantifies myocardial deformation over the cardiac cycle in radial, longitudinal and circumferential direction. Derived parameters are strain, displacement, velocity and twist that can be determined globally or on a segmental level [55]. While the post-processing is fast and easy to use, concerns have been raised regarding inter-study reproducibility of some parameters [56]. Hence, further studies are needed to finally demonstrate the value of this tool.
DENSE and SENC
Another advanced technique for cardiac wall motion assessment is Displacement Encoding via Stimulated Echoes (DENSE), which directly encodes the displacement of the myocardium into the pixel phase. DENSE provides black-blood T1-weighted images and allows the reconstruction of displacement vector maps. These vector maps can be used to calculate cardiac strain in pixel resolution. Displacement can be assessed for any arbitrary direction, e.g. two in-plane and one through-plane direction to assess three-dimensional myocardial displacement, but only one at a time. Fast imaging techniques allow multiple directions in one breath-hold, while spatial resolution is only limited by voxel size. Signal loss due to tissue perfusion and intra-voxel dephasing however limits the encoding duration. Therefore the encoding duration has to be adapted to the encoding strength to ensure that these detrimental effects are not dominant. More advance versions of this technique include strain mapping throughout the whole cardiac cycle (cine DENSE [57]), which offers tissue tracking with a high spatio-temporal resolution.
Strain encoded MR imaging (SENC) encodes myocardial longitudinal strain from two cardiac short axis acquisition. This technique is based on the MR tagging principle, but uses tag lines parallel to the imaging plane. Evaluation of spectral peaks in the frequency domain enables calculation of the myocardial strain for the desired cardiac phase.
Blood Flow Assessment
4D Flow
Blood flow measurements based on two-dimensional phase contrast acquisitions are established tools in CMR, for instance to assess valvular heart disease or shunts. The 4D-flow technique is a further development and comprises a time-resolved three-directional phase contrast measurement [58]. Image acquisition is typically done with a navigator correcting for respiratory motion, and ECG-gating. Contrast agent and higher field strength improve the image quality. Image data can be post-processed to visualize blood flow direction and patterns. Vortex as well as helix formation can be evaluated, and complex blood transfer for instance in congenital heart disease can be visualized [59]. Furthermore, quantitative information can be extracted, such as flow velocities, volumes, wall shear stress or pulse wave velocity. Most experience has been collected with the assessment of the thoracic aorta [60, 61]. For example, patients with bicuspid aortic valve show marked differences in blood flow pattern and distribution of peak systolic wall shear stress in the ascending aorta compared to healthy controls, which may contribute to aneurysm formation [62]. Additionally, intracardiac flow and non-cardiac vessel regions have also been explored [63]. Thus, 4D flow provides a new dimension of hemodynamic information that has not been accessible until now and has a great potential to help us better understand cardiovascular diseases (Fig. 22.3).
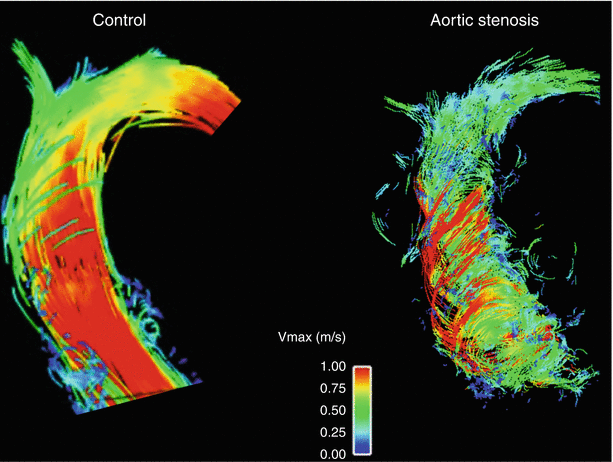
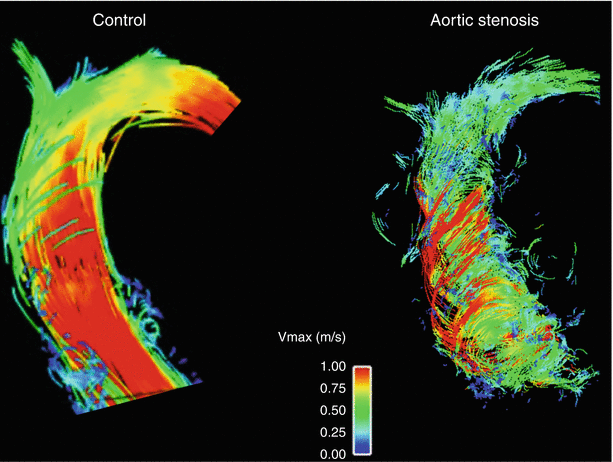
Fig. 22.3
4D-flow measurement of the ascending aorta. Compared to the healthy control (left), the patient with severe aortic stenosis showed pronounced vortex and helix formation of the blood flow
Real-Time Flow
Blood flow quantification plays an important role in many heart diseases including valvular disorders and cardiac shunts. Unlike Doppler-Echocardiography, phase contrast (PC) MRI can assess blood flow from any direction and independently from the patients’ anatomy. Similar to functional cardiac cine imaging, conventional PC-MRI collects segmented data over several heartbeats, which requires a regular heart rhythm. Fast MR sequence concepts together with accelerated data acquisition schemes were introduced that enable flow quantification in real-time without the need for splitting the data into segments [64, 65].
These concepts are promising for the quantification of blood flow in arrhythmic or uncooperative patients, as the data originates from the individual heartbeats rather than being averaged over several heartbeats. Furthermore, the real-time concept allows for the assessment of intra-individual changes in blood flow. Early techniques were challenged by a limited spatial and temporal resolution. As data acquisition acceleration techniques and scanner hardware improve, especially RF receiver coils and magnetic field gradients, faster data acquisition allows for increased spatio-temporal resolution [66].
Ultrahigh Magnetic Field Strength
CMR at 3 Tesla is now routinely performed in clinical settings. CMR at 7 T has several challenges and requires dedicated coil development, sequences development and understanding the potential safety issues.
Higher magnetic field strengths come along with an intrinsic signal-to-noise gain, which is proportional to the strength of the static magnetic field. The key driving force to strive for higher magnetic fields is this inherent advantage. The increased signal-to-noise ratio may be exploited for better spatial and temporal resolution. In human brain, histology-like images are being acquired and new techniques are about to extend the current knowledge in different disorders [67]. Also orphan diseases are likely to experience a more in depth understanding [68].
As the field strength increases, also the Larmor frequency increases and so the wavelength of the radio frequency pulses used for MRI shorten. At 7 T, the RF wavelength in cardiac tissue is approx. 13 cm (compared to approx. 54 cm at 1.5 T), which is already in the range of the human heart size. The resulting wave length effects evoke interferences causing local amplification or weakening of the transmit RF field, which finally result in heterogeneous flip angle distributions and thus heterogeneous signal intensities that can markedly deteriorate image quality at ultra-high fields.
Another challenge for cardiac imaging at ultra-high magnetic fields is the increased energy deposition in tissue, which can lead to local tissue heating. Traditional RF transmit body coils used at clinical magnetic field strengths are facing limitations at higher fields. Rather, local multi-channel transmit RF coil concepts with higher transmission efficiency are pursued that are less affected by this limitation. Additionally, multi-channel coils enable RF-shimming, a procedure to improve homogeneity of the RF transmit field by modulating RF pulse amplitudes and phases for each channel.
To overcome these problems for cardiac ultrahigh-field MRI, RF coils tailored for cardiac imaging are needed. Various RF coil designs with initial experiences have been presented by numerous groups [69, 70]. Regarding cardiac morphology and function assessment, results at 7 Tesla using spoiled gradient echo sequences are not only comparable to the 1.5 T gold standard that uses balanced steady-state free precession sequences, but also allows higher spatial resolution while maintaining diagnostic image quality.
Cardiac triggering using conventional ECG is difficult at higher field strengths due to the magneto-hydrodynamic effect. Alternative gating approaches like acoustic triggering helps to overcome this and enable robust cine imaging [71].
Despite all the expected problems first cardiac images were published in 2009 [72]. In 2010, von Knobelsdorff et al. published the first larger group of volunteers showing that cardiac chamber quantification of the left ventricle is reliable and applicable using very thin slices [73] (Fig. 22.4). Similar results were published by Suttie et al. in 2012 [74]. One would expect that the right ventricular imaging could also benefit from high resolution to characterize its thin free wall. Initial results of RV imaging at 7 T were recently published by our group [75].
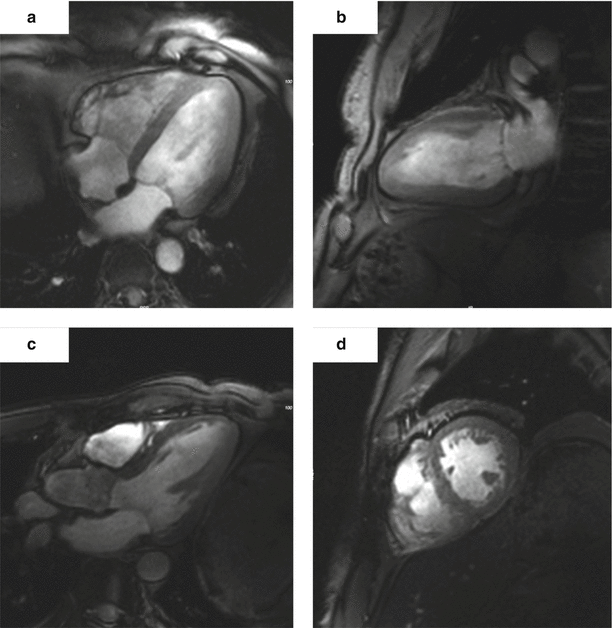
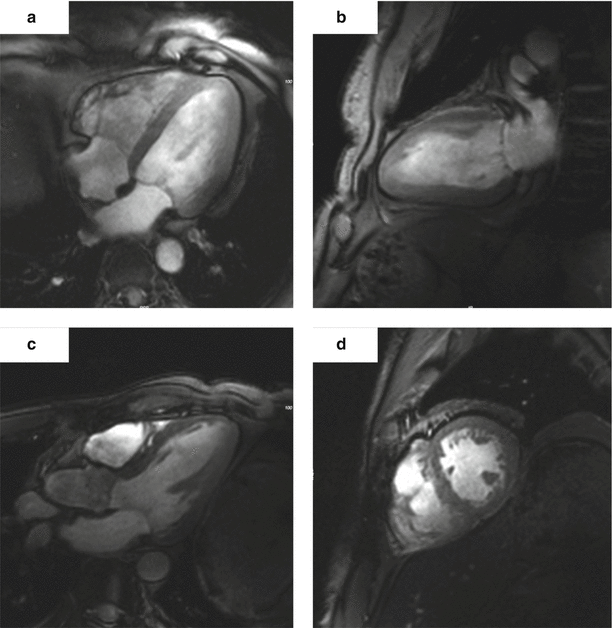
Fig. 22.4
Gradient echo cine images of the heart of a healthy volunteer obtained at 7 T. (a) Four-chamber view. (b) Two-chamber view. (c) Three-chamber view. (d) Short-axis view (The images were obtained at the Berlin Ultrahigh Field Facilty (B.U.F.F., head Prof. Thoralf Niendorf))
However, these “bread and butter” applications should only be considered the first step. The exciting promise is the step towards myocardial tissue characterization. Quantification of the myocardial deoxygenation was already tested at 1.5 Tesla, but the application at 7 Tesla seems to detect an oxygenation gradient within the myocardium [76, 77]. Myocardial spectroscopy is one of the interesting applications in magnetic resonance, as it allows for the understanding of metabolic variations. Rodgers et al. were the first to apply phosphor-MRS in the heart. [78] Fat imaging has been performed at 7 T using a 16-channel coil [79]. Another exciting recent application is the non-contrast MRA in the lower extremities showing the superiority of 7 Tesla in this first study [80].
New applications are one aspect of the current research. Simultaneously, safety aspects have to be reevaluated again. To give one example, a significant number of patients with CAD have one or more coronary stents. First simulations that analyze stent heating are offering encouraging results [81].
The potential of CMR at 7 T is currently not fully determined. But against the assumptions less than 10 years ago, the beating heart is visible at 7 Tesla and it offers new exciting avenues in CMR applications.
Outlook
The future in the field of CMR technology including higher field strengths, accelerated imaging, and increased temporal and spatial resolution including 3D-assessment.
Therapeutic options in most of the cardiovascular diseases continue to evolve due to changing population demographics and aging. Therefore, we need not only awareness of sex differences, but also of the ageing process. CMR has the capability to characterize myocardial changes early and to detect the interaction between the whole cardiovascular system. CMR applications in clinical routine have to be based on the development of standards including protocols and postprocessing [2, 82]. Clinicians are trained to use quantification for patient guidance – e.g. laboratory parameters. CMR has the capability to offer quantification for diffuse myocardial disease and intravascular alterations [60]. The establishment of accurate vendor independent and well proven mapping based quantification offers a step into non-invasive histological characterization of myocardial diseases.
< div class='tao-gold-member'>
Only gold members can continue reading. Log In or Register a > to continue
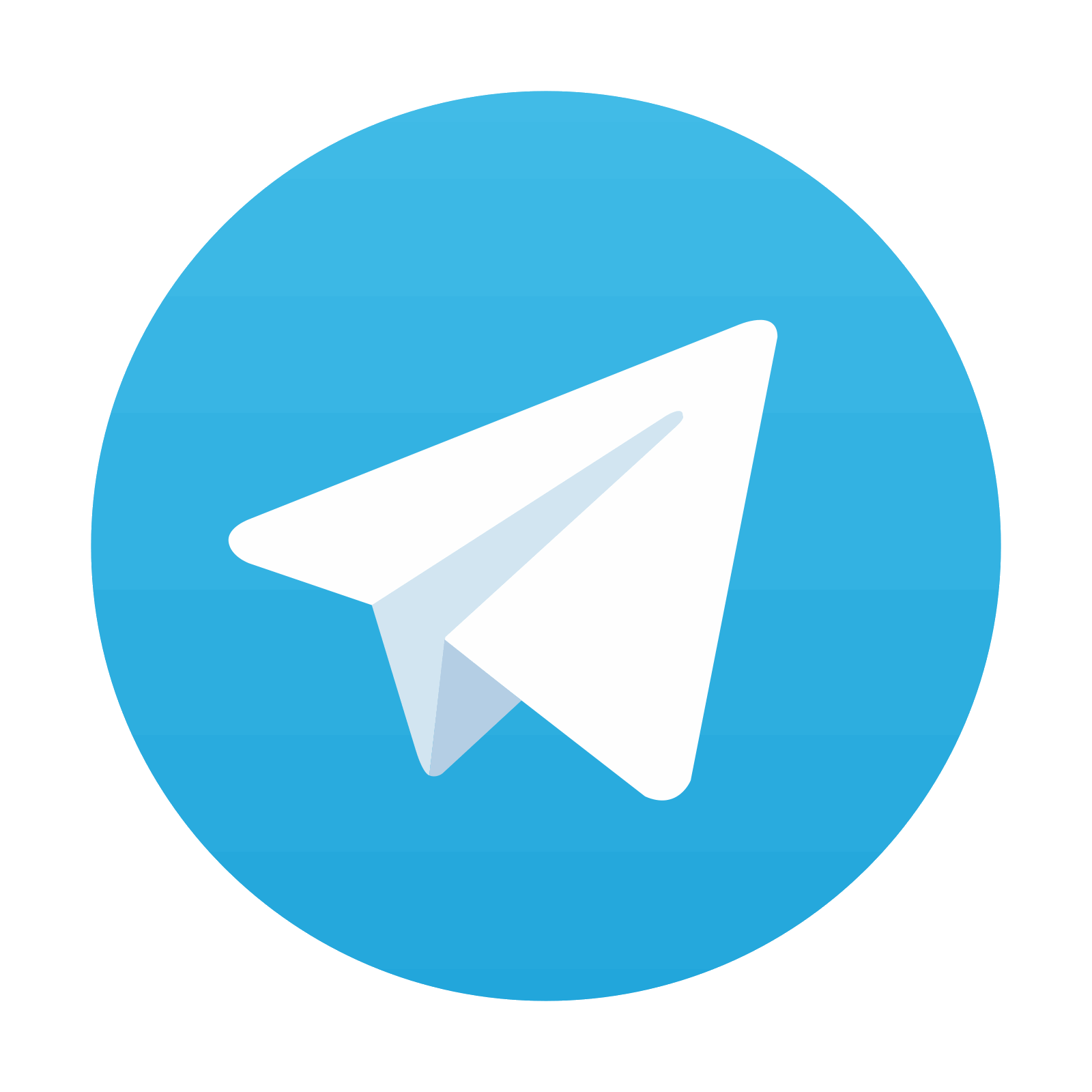
Stay updated, free articles. Join our Telegram channel
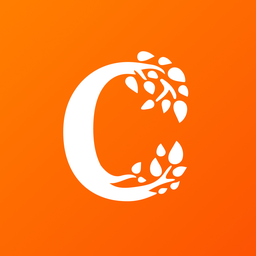
Full access? Get Clinical Tree
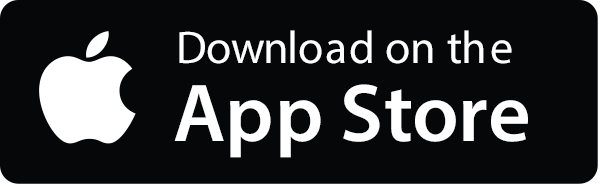
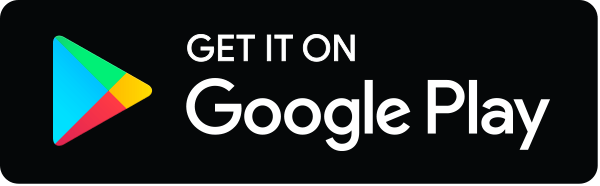