Fig. 30.1
Most common causes of early mortality after heart transplant. The most common causes of death in the first month after transplant are graft failure, neurologic events, and rejection. Infection is also a common cause of early mortality (Adapted from Kouchoukos NT et al. Kirklin/Barratt-Boyes Cardiac Surgery, 2013)
Perioperative Technical Considerations
Orthotopic heart transplantation (OHT) requires a full (often repeat) sternotomy, cardiopulmonary bypass, explantation of the diseased heart, along with any previously placed ventricular assist device(s), and allograft implantation, which may be performed using a bicaval or biatrial technique. The biatrial technique involves excision of the division of the donor left atrium anterior to the pulmonary vein ostia, incision of the right atrium from the inferior vena cava toward the right atrial appendage, and biatrial anastomosis [1, 2]. The bicaval technique is a more popular technique characterized by one left atrial and two caval anastomoses, preserving the right atrium and leaving only a small posterior portion of the recipient left atrium between the pulmonary veins [3, 4].
Intraoperative factors such as mediastinal adhesiolysis (which can be tedious and time-consuming), length of aortic cross clamping and cardiopulmonary bypass, cardiac manipulation, anesthetic factors, and other issues play an important role in the early postoperative course following reoperative cardiac surgery and OHT is no exception [5]. Great care must be taken to minimize the insults associated with each of these factors to prevent significant intraoperative and postoperative bleeding. Failure to do so may result in stroke (ischemic or hemorrhagic), renal failure, liver failure, and other systemic sequelae. A thorough discussion of the evaluation and management of postoperative stroke is beyond the scope of this chapter. Bleeding also leads to hemodynamic instability, need for massive transfusion, re-exploration, and prolonged intubation and ICU stay. Furthermore, the combination of these factors increases the risk of deep sternal wound infection (DSWI) with or without mediastinitis, discussed later [6].
Hemodynamic Complications
Allograft Dysfunction
Primary Graft Dysfunction
Primary graft dysfunction (PGD) is a phenomenon of circulatory insufficiency following orthotopic heart transplantation (OHT) caused by single ventricular or biventricular systolic graft dysfunction in the absence of rejection or other obvious etiology in the first 24–48 h postoperatively, causing hemodynamic instability requiring aggressive pharmacologic and/or mechanical circulatory support (MCS). Long considered a diagnosis of exclusion, a 2013 International Society of Heart and Lung Transplantation (ISHLT) conference created the first consensus definitions of primary and secondary graft dysfunction. Secondary graft dysfunction occurs in the context of a known cause of graft dysfunction , such as acute/hyperacute rejection, surgical complications, or pulmonary hypertension [7]. The consensus definitions and severity scale (◘ Table 30.1) of PGD are listed below:
Table 30.1
Definition and severity scale in primary graft dysfunction (PGD)
PGD-left ventricle (PGD-LV) | Mild PGD-LV: one of the following criteria must be met | LVEF < 40 % by echocardiography |
Hemodynamics with RAP > 15 mmHg, PCWP > 20 mmHg, CI < 2.0 L/min/m2 (lasting more than 1 h) requiring low-dose inotropes | ||
Moderate PGD-LV: must meet one criterion from I and another criterion from II | I. One criteria from the following | |
LVEF < 40 % by echocardiography | ||
Hemodynamics with RAP > 15 mmHg, PCWP > 20 mmHg, CI < 2.0 L/min/m2 (lasting more than 1 h) requiring low-dose inotropes | ||
II. One criteria from the following | ||
(a) High-dose inotropes—inotrope score > 10a | ||
(b) Newly placed IABP (regardless of inotropes) | ||
Severe PGD-LV | Dependence on left or biventricular mechanical support including ECMO, LVAD, BiVAD, or percutaneous LVAD. Excludes requirement for IABP | |
PGD-right ventricle (PGD-RV) | Diagnosis requires either both a and 2 or 3 alone | (a) Hemodynamics with RAP > 15 mmHg, PCWP > 15 mmHg, CI < 2.0 L/min/m2 |
(b) TPG < 15 mmHg and/or PASP < 50 mmHg | ||
(c) Need for RVAD |
- 1.
Severe left, right, or biventricular systolic dysfunction detected by direct intraoperative visualization or by measurement of left ventricular ejection fraction (LVEF) of <45 % by transthoracic (TTE) or transesophageal (TEE) echocardiography performed during or immediately after surgery
- 2.
Hemodynamic instability lasting longer than 1 h characterized by systolic blood pressure (SBP) < 90 mmHg or cardiac index (CI) < 2.2 L/min/m [2] requiring two or more inotropes or MCS with extracorporeal membrane oxygenation (ECMO), intra-aortic balloon pump (IABP), or temporary ventricular assist device (VAD) support with or without an inability to wean from cardiopulmonary bypass (CPB) despite adequate cardiac filling pressures (CVP > 15 mmHg and/or PAWP < 20 mmHg)
- 3.
Occurs within 24–48 h following implantation
- 4.
Retrospective analysis of PGD is challenging because a consensus definition was created only recently, but factors predictive of PGD can be grouped into three main groups: those related to the donor, to the recipient, or to the technical aspects of the procurement and implantation.
Donor risk factors include female sex, diabetes, head trauma, abnormal echocardiography (reduced LVEF and/or wall motion abnormality), and high-dose inotrope/vasopressor therapy prior to donation [10, 12–15]. Increased donor age is also considered a risk factor, although this is somewhat controversial [10, 12, 16, 17]. Other donor risk factors include substance abuse, preexisting myocardial or valvular dysfunction, sepsis, and hypernatremia [7].
Recipient risk factors for PGD include advanced age, need for preoperative mechanical circulatory support, heart failure due to congenital heart disease, redo sternotomy, associated left ventricular assist device (LVAD) explant, and acute and chronic medical comorbidities, including multisystem organ failure or dysfunction [7, 12, 14, 18]. Procedural risk factors include prolonged (>2–4 h) graft ischemic time, limited institutional experience, type of preservation solution, and emergent transplantation [18–22].
Allograft myocardial injury can occur as early as the onset of donor brain death or as late as reperfusion at the time of implantation. The most frequent cause of PGD is myocardial stunning secondary to inadequate myocardial protection, which can occur during the pre-procurement, transport, and implantation phases of OHT. Stunned myocardium is characterized by a period of prolonged but reversible postischemic ventricular dysfunction. The major mechanisms implicated in the etiology of stunning are intracellular calcium (Ca2+), reperfusion injury, and oxidative stress induced by reactive oxygen species, cellular swelling, extracellular edema, cellular acidosis, depletion of metabolic substrate, and endothelial injury [23]. Although stunned myocardium is viable, it is hypocontractile despite normal or near-normal coronary blood flow. Recovery may take as long as 1–2 weeks, depending on the severity and length of the ischemic insult. Currently, heart preservation after procurement is limited to 4–6 h of cold ischemic storage; longer periods of ischemia are known to adversely affect survival [24].
The current standard of care for allograft preservation in OHT is static cold storage in a slush of ice and specialized preservation solution, which is preceded by a cold flush at the time of procurement. Several such solutions, including University of Wisconsin (UW) and Celsior, are currently in use. Major features of these include high concentration of potassium and oncotic, antioxidant, and buffer additives intended to mitigate allograft edema, reperfusion injury, and acidosis. Studies examining the comparative effects of different preservation solutions on allograft function have not demonstrated one to be clearly superior [21, 24–26].
Cooling alone reduces glucose utilization, adversely alters intracellular pH regulation, and slows tissue oxygen uptake. Mitochondrial respiration and membrane integrity is reduced as well, which may cause a decline in levels of mitochondrial ATP and subsequent cellular electrochemical derangement through impaired function of ion transport channels. Although cold storage causes a dramatic decrease in myocardial energy consumption, the residual metabolic activity (~10 % of warm activity) in the absence of oxygen and glucose supply promotes anaerobic metabolism and subsequent lactic acidosis, which can be deleterious to allograft function following implantation [24].
Segovia et al. have described the RADIAL score, the first and only validated predictive scoring system for PGD based on donor, recipient, and procedural factors. Elements of the score are recipient Right atrial pressure ≥10 mmHg, recipient Age ≥60 years, recipient Diabetes mellitus, recipient Inotrope dependence, donor Age ≥30 years, and Length of ischemic time ≥240 min. Each donor-recipient pair receives a single point for each element present. Further work demonstrated that the RADIAL score can be used to create three risk strata, low (RADIAL < 2, PGD risk 12.1 %), intermediate (RADIAL = 2, PGF risk 19.1 %), and high (RADIAL > 2, PGD risk 27.5 %).
The cornerstone of management of PGD is mechanical circulatory support (MCS) . MCS in patients with PGD can be provided using intra-aortic balloon pumps (IABP), extracorporeal membrane oxygenation (ECMO), and temporary ventricular assist devices (VADs). Most data regarding the use of these devices for PGD is retrospective in nature [9, 19, 27, 28]. Algorithms for the use of MCS , and its concomitant use with pharmacologic support for PGD, are generally individualized between high-volume centers [29]. There is a trend toward early initiation of MCS, frequently prior to leaving the operating room, with the goal of mitigating the systemic insult of a period of prolonged cardiac dysfunction [11].
Inotropes, vasodilators, and inhaled nitric oxide serve as valuable adjuncts in this setting, although no universal protocol exists for their use. Levosimendan, one of the only agents for which dedicated data exists in this setting, is a calcium channel sensitizer that acts as a positive inotrope and peripheral vasodilator without increasing myocardial oxygen demand and has been specifically studied for use in PGD. A small descriptive case series of heart transplant patients with PGD treated with levosimendan demonstrated expeditious weaning from other pharmacologic support and 93 % survival at 30 days after surgery, with only one patient requiring MCS [30]. However, these benefits were not appreciated at 3 years of follow-up [31].
Heart transplantation carries a 30-day mortality of approximately 8 %, during which time PGD is responsible for 35–40 % of deaths, making it the leading cause of early mortality following heart transplant [32]. The mortality risk of PGD managed exclusively with pharmacologic means is 40–50 % [33]. In some series the use of ECMO for PGD is associated with 30-day and 1-year survival as high as ~80 % and 70 %, although the need for VAD support portended worse overall outcomes [33].
Right Ventricular Failure
Risk Factors
Defined as a failure of the right ventricle (RV) to maintain adequate flow through the pulmonary circulation to allow adequate LV filling, right ventricular failure remains a significant and vexing problem after cardiac transplantation and a source of major morbidity and mortality in the perioperative period [34]. Severe RV failure is defined by ballooning of the RV with corresponding end-organ dysfunction or the need for mechanical circulatory support with a right ventricular assist device (RVAD) [35].
The most common risk factor for RV failure following OHT is ischemia-reperfusion injury, which is caused by the process of organ procurement, cold ischemic transport, and implantation/reperfusion. Other risk factors include mechanical trauma during transport and implantation, warm ischemia in the operating room, coronary air embolus, and any extrinsic factor that would raise pulmonary vascular resistance, such as mechanical ventilation with high PEEP and acute and/or chronic recipient pulmonary hypertension [35]. Common causes of posttransplant RV failure are summarized in ◘ Fig. 30.2.


Fig. 30.2
Etiologies of posttransplant right ventricular (RV) failure. RV failure following transplant is generally related either to dysregulation of preload, impaired contractility due to intrinsic myocardial dysfunction or insufficient coronary flow, or excess afterload causes by left ventricular dysfunction, pulmonary vasoconstriction/hypertension, or valvular/anastomotic narrowing (Adapted from Itagaki S et al. Semin Cardiothorac Surg 24:188)
The complicated geometry of the RV makes it difficult to measure its function directly. Jugular venous distention, peripheral edema, a split second heart sound, and a tricuspid regurgitation murmur are clinical data suggestive of elevated right-sided filling pressures, especially in the absence of left-sided dysfunction. A number of echocardiographic adjuncts, such as RV size, tricuspid annular plane systolic excursion (longitudinal displacement of the RV base relative to the RV apex), and estimated RV systolic pressure, are helpful in the diagnosis of RV failure, although each of these can be affected by factors extrinsic to RV function [34]. Pulmonary artery (PA) catheters are routinely left in place following OHT and data such as central venous pressure (CVP) and PA pressure are crucial in making this diagnosis.
Management of Right Ventricular Preload and Contractility
Elements of the management of RV failure can be grouped into those modifying preload, afterload, and the intrinsic contractility of the ventricle (◘ Fig. 30.3). The RV is a thin-walled and highly compliant chamber that responds poorly to acute increases in preload. Acute distention of the RV can induce a level of wall tension beyond which it is unable to pump effectively. Furthermore, ventricular interdependence, a phenomenon in which massive RV distention can cause the ventricular septum, which forms the posterior wall of the RV, to invert its curvature and impede LV filling. Poor LV filling has a negative impact on its function, which can exacerbate right heart failure. Conversely, inadequate filling of the RV, most commonly caused by systemic hypovolemia, can also impair RV output [36]. The great sensitivity of the RV to fluid overload mandates careful management of volume status in these patients, often with the aggressive use of diuretics and, in some cases, hemofiltration [34, 36].


Fig. 30.3
Management of posttransplant RV failure . A suggested algorithm for the correction of RV dysfunction following transplant. In the absence of an obvious cause of RV failure that may or may not be immediately correctable, optimization of RV function should be considered in a stepwise manner, although simultaneous correction of multiple issues may be appropriate based on a particular clinical situation (Adapted from Ventetuolo CE et al. Ann Amer Thorac Soc 11: 811)
Decreased contractility in RV failure is considered to be due either to impaired inotropy or impaired myocardial perfusion. Impaired inotropy can be due to myocardial stunning from ischemia-reperfusion injury, mechanical trauma, and donor or recipient sepsis [35, 36]. Perfusion to the myocardium of the right ventricle can be compromised by impaired inflow to the right coronary system, which can be caused by air embolism or thromboembolism of the right coronary artery (RCA), kinking of the RCA due to extrinsic compression or problems with allograft positioning at implantation, and impaired global coronary perfusion due to LV dysfunction. Impaired RV contractility is managed primarily with inotropes, such as dobutamine, milrinone, and isoproterenol. Isoproterenol is customarily used for several days after OHT for its positive chronotropic effects, although there are no prospective studies comparing its effectiveness in this regard to other inotropes [37, 38].
Right Ventricular Afterload and Pulmonary Hypertension
Right ventricular afterload is dictated by the pulmonic valve and the resistance of the pulmonary vascular bed. Pulmonary vasoconstriction, which can elevate pulmonary vascular resistance (PVR) can be caused by hypoxia, hypercarbia , acidosis, massive transfusion, and protamine administration, all of which can be common at the time of OHT [36]. Extrinsic compression or other compromise of pulmonary inflow caused by pneumothorax, massive pleural effusion, pulmonary embolus, and elevated intrathoracic pressures caused by positive-pressure ventilation with high PEEP can also increase PVR [36]. Complications associated with the PA anastomosis, such as narrowing or kinking, can create a fixed mechanical impediment to RV unloading and must be, along with the other factors listed above, avoided or corrected at the time of implantation.
Recipient pulmonary hypertension (PH), defined as invasively measured mean pulmonary artery pressure ≥25 mmHg, is associated with high morbidity and mortality in the acute postoperative period after OHT [35]. Chronic elevation of left-sided filling pressures resulting from LV systolic dysfunction is the most common cause of pulmonary hypertension in OHT recipients [39]. Other common causes of pulmonary hypertension include chronic hypoxia (e.g., COPD, sleep apnea, and developmental/interstitial lung disease), chronic pulmonary thromboembolic disease, and secondary effects of many systemic illnesses, such as chronic kidney disease, sarcoidosis, and hemolytic anemias. Pulmonary arterial hypertension, which can be idiopathic or associated with other comorbid conditions such as scleroderma or portal hypertension, is rare in OHT recipients.
Parameters such as transpulmonary gradient (TPG, mean PA pressure-mean pulmonary capillary wedge pressure, mmHg) and pulmonary vascular resistance (PVR,
,
), which is frequently described in Wood units (
), are generally obtained with the use of a PA catheter and are useful in characterizing the severity of pulmonary hypertension [40]. At the time of measurement, patients are treated with short-acting pulmonary vasodilators to determine the reversibility of their PH. Nonreversible PH is predictive of early and late mortality after OHT because of the attendant risk of RV failure. It is generally agreed upon that a resting PVR > 5 Wood units is a contraindication to OHT, although, while mortality in this setting is strongly linked to increasing severity of PH, no threshold level of PVR is absolutely predictive of RV failure [40, 41]. Patients with PH in whom PVR can be reduced to ≤2–3 Wood units with the use of inhaled or intravenous pulmonary vasodilators prior to OHT demonstrate a mortality risk essentially equivalent to heart recipients without preexisting PH [42–45]. There may, however, be an elevated risk of post-op RV failure, and patients who have residual PH after transplant may have decreased long-term survival [42, 43].



Implantable long-term left ventricular assist device (LVAD) therapy has been used to ameliorate fixed pulmonary hypertension in patients with end-stage heart failure being considered for OHT [46–48]. Maximum benefit appears to be reached by 6 months of therapy, with decreases in TPG and PVR predicting the greatest mortality benefit after OHT compared to patients who did not receive pretransplant MCS [46, 47].
Postimplantation RV failure is commonly recognized in the operating room based on the presence of a distended and hypokinetic RV, underfilling of the LV , and/or elevated PA pressures; these can be detected by transesophageal echocardiography, direct visualization, and data from the PA catheter [49]. A low threshold should be held for the initiation of pulmonary vasodilator therapy, either with the use of either inhaled nitric oxide or prostacyclin, which are equally efficacious in this setting [50]. Both of these medications require gradual weaning because of the potential for rebound PH with rapid withdrawal of therapy. Sildenafil, commonly started either before or in the days following transplant, is also effective in combating recipient PH [51]. Slow (days to weeks) weaning of inotropic medications such as milrinone and/or dobutamine and efforts to minimize PEEP in the postoperative period can also be important adjuncts following transplantation in patients with pulmonary hypertension and/or any evidence of RV failure [36].
Mechanical Circulatory Support
Frequently, mechanical circulatory support (MCS) is required in the management of posttransplant RV failure that is refractory to maximal medical therapy. IABP can be useful in the setting, with one series demonstrating near-immediate improvement in systemic and right-sided hemodynamics following IABP insertion with durable response and pump removal after an average of ~44 h of therapy [52]. More severe cases of posttransplant RV failure have required the use of right ventricular assist devices (RVADs) or biventricular assist devices (BiVADs).
Multiple case reports and series from the late 1980s through 2000 report the use of extracorporeal RVAD support immediately following OHT in a total of 20 patients [53–59]. The most common indication for MCS in this aggregate cohort was recipient pulmonary hypertension. Outcomes in these studies were almost universally poor; only three studies reported a total of four patients who survived to discharge [53–55]. Of the patients that died, nine were able to be weaned from RVAD support (56 %) before death. The most common causes of death among all non-survivors were sepsis, multisystem organ failure, and hemorrhagic complications [53, 54, 56–59].
In 2003 Kavarana et al. described a series of 20 heart recipients who received postoperative MCS , of whom 14 required either RVAD or BiVADs. Consistent with prior studies, the most common indication for support was recipient pulmonary hypertension. Of all RVAD/BiVAD recipients in this study, only six (43 %) could be weaned from support, and two of those patients went on to die of multisystem organ failure. Of those who did not wean, the most common causes of death were intraoperative arrest, refractory heart failure, and sepsis [9]. More recently, Klima et al. reported in 2005 a series of 35 patients who met criteria for severe RV failure following OHT and 15 of these patients received some combination of RVAD, ECMO, and IABP; no mortality benefit was realized from the use of MCS in this cohort [35].
Overall, the need for MCS for RV failure following heart transplantation portends extremely high mortality. Although recovery to discharge is possible, including after as long as 80 days of RVAD therapy in one case report, the incidence of fatal complications is extremely high in this cohort, and a frank and thoughtful discussion should occur between all involved providers and loved ones before this level of support is initiated [60].
Heart Size Mismatch
Donor-recipient size matching is an established component of the organ allocation process. Traditionally, a weight difference of <20 % has been sought in pairing donors and recipients for OHT, but outcome data over the last two decades have undermined this practice. It has been demonstrated previously that weight and heart size are not meaningfully correlated [61, 62]. A retrospective, single-center study in the early 1990s demonstrated no increase in mortality with a weight differential up to 30 % and another such study demonstrated increased mortality from undersized donors only in recipients who were listed United Network for Organ Sharing (UNOS) status I for transplant [63, 64].
A multicenter retrospective analysis of UNOS data in 2008, the largest study of this question to date, demonstrated that donor-recipient weight differentials of >20 % did not affect survival, except in a few special circumstances. The implantation of undersized hearts in recipients with significant pulmonary hypertension negatively affected survival, particularly when a female donor was paired with a male recipient. Interestingly, no survival advantage was conferred by the use of oversized allografts in recipients with PH [65]. Recent data from the Multi-Ethnic Study of Atherosclerosis (MESA) study have shown that heart size does not depend only on weight but also on height, age, and sex, suggesting that it may be more appropriate to size match donors and recipients on these data in lieu of weight alone [66, 67].
Rejection
Hyperacute Rejection
Immediate allograft failure caused by binding of preexisting recipient antibodies to donor vascular endothelium by preexisting recipient antibodies is known as hyperacute rejection . This immunologic reaction occurs within minutes to hours after restoration of allograft perfusion and results in complement activation and intravascular thrombosis. Lysis of capillary endothelial cells leads to disruption of vessel integrity, loss of vascular contents, and exposure of platelets to the underlying matrix resulting in platelet adhesion, aggregation, and local vasoconstriction due to loss of nitric oxide. This is ultimately followed by interstitial hemorrhage without lymphocytic infiltrate [68, 69]. Largely prevented by ABO and human leukocyte antigen (HLA) matching, the only therapy for this catastrophic diagnosis is emergent MCS therapy and retransplantation, although outcomes are extremely poor even if a suitable organ can be found [70].
Acute Cellular Rejection
Prior to the development of cyclosporine in the 1970s, inability to manage rejection safely and reproducibly was the primary barrier to the growth of heart transplantation following its early development [71]. Although rejection rates continue to decline, the risk of rejection remains significant, particularly in the early period following transplantation, necessitating routine surveillance for both acute cellular (ACR) and antibody-mediated rejection (AMR).
ACR is characterized by a predominantly T-cell-mediated response with infiltration of lymphocytes and macrophages into the interstitium of the allograft, which may lead to myocyte necrosis [70]. Although it can occur at any time after OHT, it occurs most commonly in the first 3–12 months after transplant. Nearly 40 % of adult heart transplant patients have one or more ACR episodes to some degree within the first month, and over 60 % experience one or more ACR within 6 months. At 1 year only one third of patients remain free of ACR. Within the first year, about 30 % of patients will have rejection which will require adjustment of immunosuppressive therapy. Risk of ACR beyond 1 year is low and does not appear to change over time [72, 73].
Donor risk factors for ACR within 1 year of transplant include female sex, positive CMV serology, brain death, and ischemia-reperfusion injury. Brain death and ischemia-reperfusion are thought to increase donor antigen expression, which may promote ACR. Recipient risk factors include female sex, young age, African-American race, increased HLA mismatching, prior infection following transplant, and recent (separate) episodes of rejection [74]. The development of acute rejection requiring treatment predicts a higher incidence of cardiac allograft vasculopathy and long-term mortality [75, 76].
In part because of the allograft denervation inherent to the transplant process, the presentation of ACR can be protean in nature, manifesting as any combination of fever, leukocytosis, mild hypotension, or other vague systemic symptoms [77]. In rare cases, the presentation is much more dramatic, with patients demonstrating severe heart failure, refractory hypotension, or circulatory collapse [69]. The inconsistent presentation of ACR makes endomyocardial biopsy (EMB) the gold standard for diagnosis. The biopsies are obtained percutaneously, usually from the interventricular septum, with a dedicated bioptome passed through the internal jugular or femoral vein [69]. Pathologic analysis of the biopsy specimens is used to grade the severity of ACR in accordance with ISHLT guidelines, which were most recently updated in 2004. ACR is graded on a scale from 0R (no evidence of ACR) to 3R (severe ACR) based on the level of cellular infiltrate and tissue destruction (myocyte damage, edema, hemorrhage, and vasculitis) (◘ Table 30.2) [78].
Table 30.2
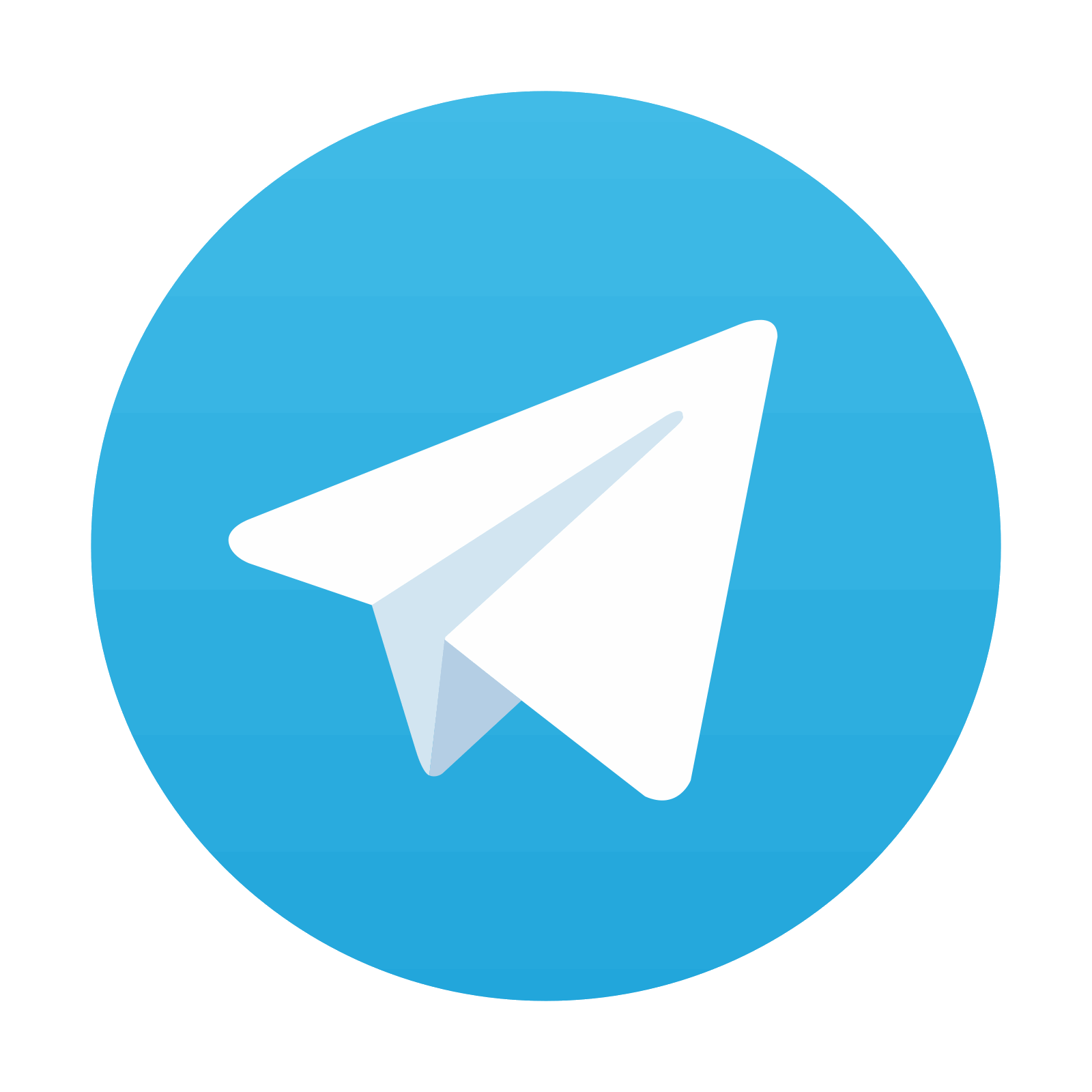
ISHLT biopsy grading for acute cellular rejection (ACR)
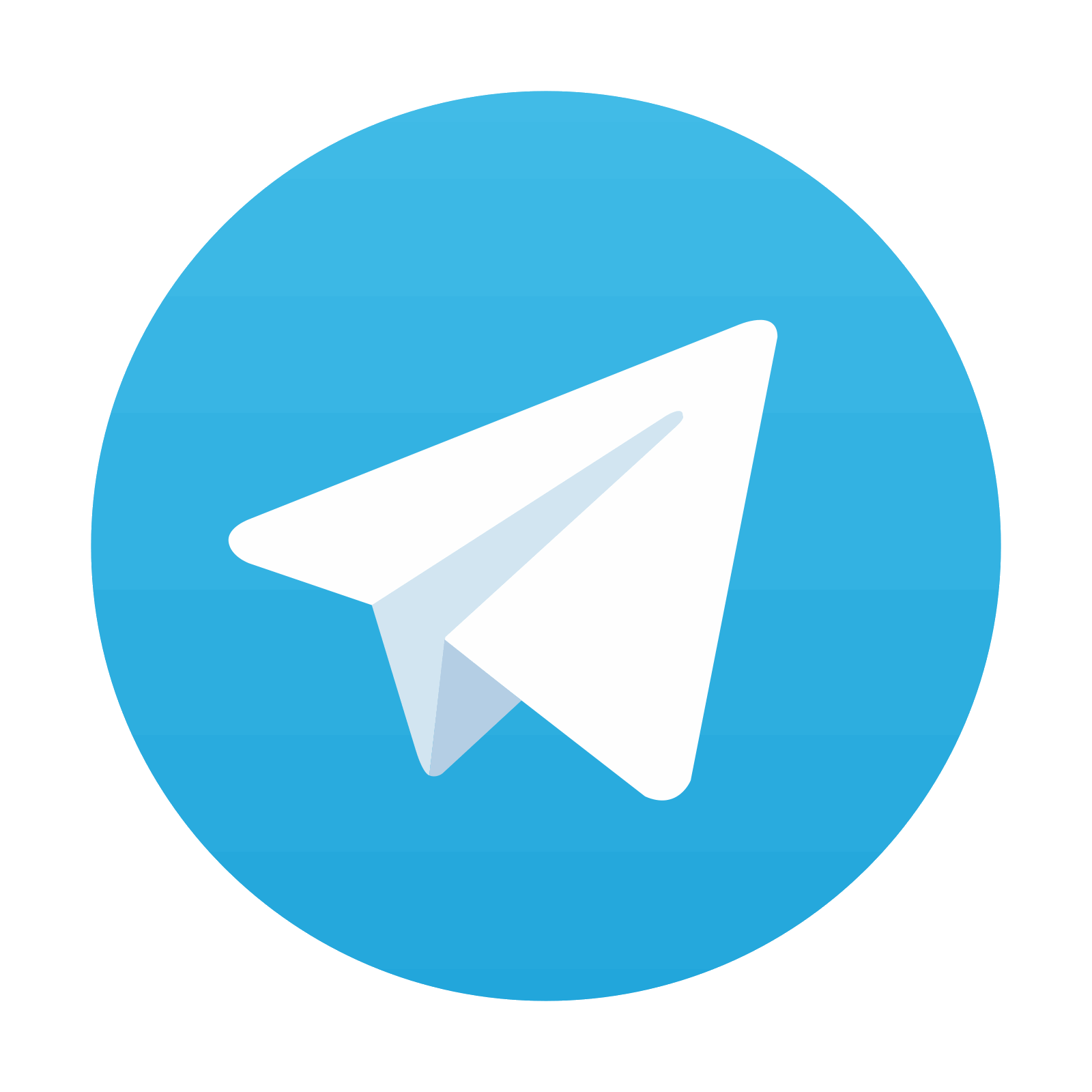
Stay updated, free articles. Join our Telegram channel
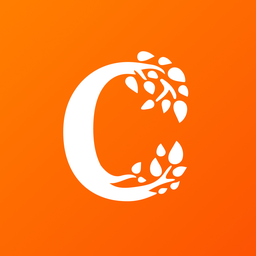
Full access? Get Clinical Tree
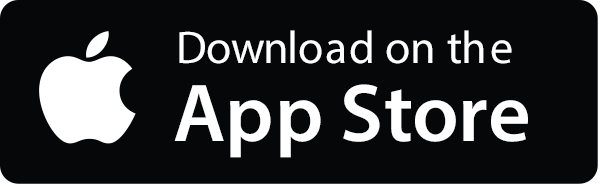
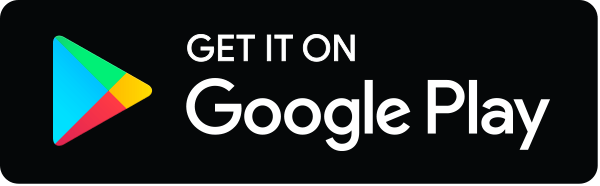
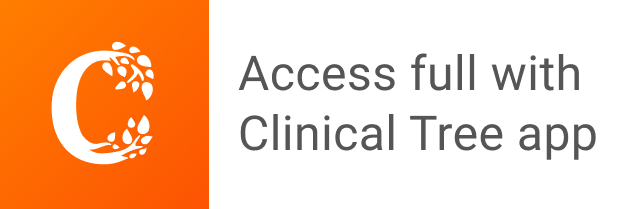