Fig. 9.1
Mechanisms of acute RV failure. Acute RVF frequently is multifactorial and includes a number of pathological conditions that result in reductions in RV contractility and preload, in addition to increases in RV afterload. Note that several mechanisms may cause acute RV failure by two or more of these components. Asterisks and hashtags indicate mechanisms not directly demonstrated in the schematic: *Pulmonary microthrombi can also be seen in sepsis/SIRS. #Sepsis/SIRS may cause acute pulmonary hypertension due to pulmonary artery endothelial cell dysfunction. **LV dysfunction may also increase preload. ##Valvular heart disease may also affect RV function by increasing afterload (e.g., pulmonary stenosis). ARDS acute respiratory distress syndrome, IL-1β interleukin-1β, IL-6 interleukin-6, LV left ventricle, TNF tumor necrosis factor
Preload dependence: The RV is perfectly adapted to generate an adequate and appropriate cardiac output (CO) into the low-pressure pulmonary circulation [7, 25]. As a result of its high compliance, the RV is well adapted to accommodate sudden and significant changes in preload [25–27]. In fact, it is the premier function of the RV—to guarantee a sufficient amount of blood flow through the pulmonary vasculature and into the LV, in order to allow for the required changes in CO in the setting of positional changes, exercise, or disease. However, even though the RV is custom-built to accommodate large changes in preload, at either end of the Frank–Starling curve (significant underfilling or overdistension), the RV can lose this capacity. Acute RVF from underfilling may occur during hypovolemia, sepsis, superior vena cava obstruction (e.g., SVC syndrome), mechanical ventilation (MV), or arrhythmias. On the other hand, RV failure from overfilling and overdistension can occur during hypervolemia or overzealous volume resuscitation. Preload sensitivity is particularly pronounced in the setting of increased pulmonary vascular resistance (PVR), including embolic syndromes, hypoxic pulmonary vasoconstriction (HPV), or mechanical ventilation [2, 8].
Contractility: The RV acutely responds to afterload stress by enhancing contractility via the Frank–Starling mechanism, the Anrep mechanism (see Chap. 2), and neurohormonal activation [8, 25, 28]. RV contractility can be reduced acutely as a consequence of impaired energy metabolism, cytokine activation, and/or endotoxin release during sepsis, acidosis, ischemia, electrolyte disturbances, drug overdose (e.g., beta blockers, calcium channel blockers), or substrate depletion [2, 5, 8, 29, 30]. Several of these processes may simultaneously affect LV function, thus leading to biventricular failure.
Afterload sensitivity: While the RV responds well to demand for volume work from preload increase, the RV responds poorly to pressure work demand from increased afterload [8, 26, 31–34]. This principle is particularly important in the setting of acute afterload increase; acute PE is the prototype of such an insult. In general, a previously healthy and non-hypertrophied RV cannot acutely overcome a systolic pulmonary arterial pressure (PAP) of more than 50–60; it will decompensate [2, 8, 35]. Consequentially, any condition that leads to a significant loss of pulmonary vascular cross-sectional area (e.g., acute embolism syndromes, severe acute PH, acute HPV, pulmonary microthrombi in sepsis, or ARDS) may result in profound RVF, as the RV will not be able to generate a high enough PAP to maintain pulmonary vascular perfusion and CO. On the other hand, in the setting of a chronically elevated afterload, the presence of RV hypertrophy allows for the RV to generate much higher pulmonary pressures, making it more resilient to afterload stress [8]. Even though RV contractility initially increases as a compensatory response to afterload stress, if the rise in contractility is not sustained or sufficient for the degree of afterload increase, this results in RV–PA uncoupling [36] (see Chap. 2). Pressure–volume measurements in the RV allow for accurate measurements of RV contractility and RV–pulmonary artery (PA) coupling in the setting of RV afterload stress, in the research setting [36–38].
Ventricular interdependence and dependence upon perfusion during systole also contribute to the RV’s vulnerability. While alterations in preload, contractility, or afterload usually initiate acute RV dysfunction, ventricular interdependence and a drop of the perfusion pressure are causes of rapid RV failure. Ventricular interdependence is due to three distinct phenomena: (1) the connection of RV and LV superficial muscle layers in the ventricular groove, (2) the shared interventricular septum (IVS), and (3) the poorly compliant pericardial sac limiting the expansion of one ventricle in expense of the other ventricle’s filling [39–41]. Due to connected superficial muscle layers and shared IVS, LV contraction contributes to roughly 20–40 % of the RV systolic pressure (RVSP) and ejection fraction [42–44]. In acute RV pressure- or volume overload however, both distortion of the RV shape and leftward shift of the IVS significantly compromise the contractile contributions of the LV to RV function [8, 39, 40, 42]. Importantly, due to constraints of the pericardial sac, the “bowing” of the IVS towards the LV also impinges on that ventricle, leading to LV underfilling and diastolic dysfunction, thus further impairing overall CO [39, 42, 45]. Similarly, ventricular interdependence can lead to acute RV dysfunction in the setting of LV failure, with the IVS being shifted to the right, and thus impinging on the RV [46].
RV perfusion: Muscle ischemia plays a difficult to assess role in the development of acute RVF. While RV coronary perfusion under normal conditions is characterized by coronary flow during both systole and diastole [38, 47, 48], with increasing wall stress and a subsequent rise in myocardial transmural pressure, coronary perfusion via the right coronary artery (RCA) is reduced, leading to an overall decrease in oxygen delivery and subsequent regional ischemia with the subendocardium most severely affected [49–52]. This decreased oxygen delivery is further compounded by an increase in myocardial oxygen demand secondary to increases in both RV wall stress and workload [50]. The tachycardia that is frequently observed in the setting of RV dysfunction further contributes to decreased oxygen delivery and increased oxygen demand. In the chronically dysfunctional RV, remodeling may promote adaptive changes in the microvasculature with increased capillarization and compensatory biochemical and molecular changes [30, 53]. However, in the setting of acute RV dysfunction or failure, these adaptive mechanisms are not available [53]. As a result, profound myocardial ischemia ensues leading to impaired RV contractility. This reduction in contractility further impairs overall CO and consequently reduces oxygen delivery to an already substrate-deprived RV, leading to a viscous cycle until its ultimate collapse (Fig. 9.2). Many authors believe that it is the myocardial ischemia that accounts for the ultimate transition from RV dysfunction to failure [30, 51, 53].


Fig. 9.2
Vicious cycle in the transition from RV dysfunction to failure. Alterations in preload, increases in afterload, and/or decreases in contractility are the causative factors in the development of RV dysfunction. If the stimulus is severe and/or sustained, increased wall stress and increased oxygen demand will result in local ischemia. Systemic hypoxemia caused directly by the underlying disease triggering the RV dysfunction (e.g., ARDS, pulmonary hypertension, sepsis, systemic inflammatory response syndromes, exacerbations of chronic lung disease, or congestive heart failure) may contribute to the development of local ischemia in the RV. Once RV cardiomyocyte ischemia ensues and contractility decreases, a vicious cycle of increased oxygen demand in the setting of decreased oxygen delivery occurs, leading to worsening RV failure and its eventual collapse. RV right ventricle, R → L right-to-left shunt
Other components of RV performance that may contribute to an acute deterioration in RV function include loss of normal sinus rhythm, loss of RV intraventricular synchrony, and loss of valvular competency [25, 45, 54, 55]. In particular, the acute onset of tricuspid regurgitation (either as a result of papillary muscle rupture in acute myocardial infarction or as a result of significant RV dilation from pressure or volume overload) can have detrimental consequences [26].
Biochemical and Molecular Changes in the Acutely Failing RV
Biochemical and molecular correlates of acute RVF include cardiomyocyte death, influx of pro-inflammatory cells, chemokine/cytokine activation, and oxidative stress. Cardiomyocyte death is a consequence of ischemia, substrate depletion, and impaired mitochondrial energy metabolism. Intracellular calcium overload and oxidative stress are additional contributors to cell death. Significant biochemical alterations are observed within 24 h of RV injury.
PE is the prototype of acute RV injury. The abrupt increase in the afterload results in RV muscle stretch and shear stress, increased wall tension and dilation, elevated heart rate, and increased oxygen demand in the face of decreased RV coronary perfusion. The result is a poorly contractile, dilated, thinned, and pale-appearing RV [56] (Fig. 9.3a + b). Histologically, these changes are accompanied by cardiomyocyte lysis and an inflammation. Influx of neutrophils, lymphocytes, and macrophages occurs within hours of the insult [57–59] (Fig. 9.3e–g). Macrophages exhibit an M1 pro-inflammatory phenotype, and compared to neutrophils (which decrease within 1 week) they are still present at 6 weeks (then characterized by a M2 wound repair phenotype) [57]. Myeloperoxidase, MCP1, CINC1, CINC2, MIP1α, and MIP2 are activated/increased within 18 h of the insult, and are accompanied by matrix metalloproteinase activation, cardiomyocyte necrosis, and mitochondrial damage [56, 60]. Similar changes with activation of pro-apoptotic pathways and local overexpression of TNF-alpha were also seen in an acute PA constriction model, where these changes occurred within 2 h of RV injury [61]. Pro-inflammatory biomarkers (e.g., myeloperoxidase) can also be detected in the serum of patients with acute PE [62–64]. Inflammation is pathogenetically relevant, since treatment with anti-PMN or anti-CINC (CXCL1) antibodies or with the nonsteroidal anti-inflammatory drug ketorolac reduces RV inflammation and improve contractile function [56, 65, 66]. Reactive oxygen species (ROS) can be demonstrated within 24 h of acute PE [60]. The RV outflow tract is particularly affected by acute afterload stress [57, 67] (Fig. 9.3a–f). RV deformation normalizes with time in cases of less severe cases of acute PE; however, in more severe PE, RV dilation, thinning, and scarring may remain permanent, leading to persistently impaired contraction [68] (Fig. 9.3h).


Fig. 9.3
Macroscopic and microscopic correlates as well as long-term consequence of acute RV failure due to pulmonary embolism (PE). (a–d) Images of both whole heart (a, c) and RV free wall (b, d), respectively, of isolated perfused rat hearts 18 h after experimental acute PE (a, b) or sham procedure (c, d). Note the pale appearance of the RV outflow tract (RVOT) in (a) and (b), representing significant myocardial injury. (e–g) Staining for neutrophils (PMNs) in RVOT tissue from animals treated with PE at 2 h (e), 6 h (f), and 18 h (g) shows marked time-dependent infiltration with PMNs. (h) Long-term consequences of acute severe PE. Contrasted chest computer tomography (CT) image from a 79-year-old male with a severe acute PE 6 months prior. Symptoms at the time of imaging were lower extremity edema and dyspnea on exertion. CT shows a markedly dilated RV and right atrium (RA). Of note, this patient did not have any evidence of chronic thromboembolic pulmonary hypertension on ventilation/perfusion scanning and right heart catheterization. (a–d) Reproduced with permission from Watts JA et al. [56]; (e–h) reproduced with permission from Watts JA et al. [303]
Though not specifically described for the RV, experimental data suggest that cardiomyocyte stretch leads to activation of an angiotensin II- and endothelin (ET)-mediated increase in cytosolic calcium and subsequent activation of NFAT, Ca2+/calmodulin-dependent kinase II, and PKC signaling [69]. Calpain activation contributes to contractile dysfunction in acute RVF and is associated with decreased abundance and organization of the adhesion protein talin [70, 71]. Lastly, a switch to the fetal gene program similar to what is observed in chronic RVF has also been described for acute RV injury. For example, in a rat model of acute PE, a decrease in the expression of genes, encoding proteins involved in fatty acid transport, activation, and β-oxidation occurs within 18 h of injury [72]. This is accompanied by activation of hypoxia-inducible factor (HIF)-1α, suggesting that—as in chronic RVF [53]—HIF-1α activation is a key regulator of these metabolic changes.
Etiologies of Acute RV Failure
In general, the RV fails as a consequence of alterations in preload, contractility, and/or afterload [2, 8, 26]. While a separation into these three entities helps understand and identify etiologies of RV dysfunction or failure, many conditions can alter several of these components simultaneously (e.g., sepsis, which may alter preload, contractility, and afterload [11]). The following section discusses the clinically most relevant etiologies of acute RVF. A detailed overview of the various etiologies is provided in Table 9.1 and Fig. 9.1.
Table 9.1
Common etiologies of acute right ventricular failure
Preload decrease |
• Hypovolemia (over-diuresis, hemorrhage) |
• Acute systemic vasodilatation (sepsisa) |
• Mechanical ventilationa |
• SVC syndrome |
• Acute pericardial disease (constrictive pericarditis, cardiac tamponade) |
Inotropic impairment |
• Left ventricular systolic or diastolic dysfunction (via ventricular interdependence; possibly also via arrhythmias) |
• RV myocardial infarction/ischemia |
• Acute myocarditis |
• Sepsisa |
• LVAD placement |
• High-intensity exercise |
• Brain death |
• Arrhythmias |
• Metabolic derangements (electrolyte disorders, acid base disturbances) |
Afterload increase |
• Pulmonary hypertension (PH) |
– Pulmonary arterial hypertension (PAH: WHO group 1) |
– Pulmonary venous hypertension (WHO group 2) |
– PH due to chronic lung disease/hypoxemia (WHO group 3) |
– CTEPH (WHO group 4) |
– Other causes of PH (WHO group 5) |
• Acute pulmonary embolism |
• Acute embolism syndromes (Fat, amniotic fluid, cement, tumor) |
• Hypoxic pulmonary vasoconstriction (ARDS) |
• Mechanical ventilationa |
• Pulmonary vein stenosis/RV outflow tract obstruction |
• Post-cardiothoracic surgery (CABG, heart or lung transplantation, corrective surgery for CHD) |
• Acute chest syndrome |
• Pulmonary microthrombi (sepsisa, DIC, ARDS) |
• High-intensity exercise |
Complex heart diseases |
• Valvular heart disease (aortic, mitral, pulmonary, or tricuspid valve) |
• Congenital heart disease (atrial/ventricular septal defect, Ebstein’s anomaly, tetralogy of Fallot, transposition of great vessels, Uhl’s anomaly, anomalous pulmonary venous return) |
• Chronic restrictive cardiomyopathies (amyloidosis, sarcoidosis, idiopathic) |
The most common etiology underlying acute RV failure is decompensated LVF [8]. The development of RVF in LV disease is multifactorial, and includes pulmonary venous congestion, ventricular interdependence, left-sided arrhythmias, and/or myocardial ischemia [8, 29, 73].
Acute PE and acute PH are also common causes of acute RVF [2, 8, 19, 26, 35, 74]. In many ways, these two syndromes are the prototypes of acute RV dysfunction caused by an excessive increase in afterload. In the early 1970s, McIntyre and colleagues documented, using pulmonary angiography, the tight correlation between pulmonary vascular occlusion and pulmonary hypertension in patients without preexisting lung disease [75, 76]. In previously healthy patients, at least 40 % of the cross-sectional area must be obstructed to significantly increase the PAP and produce abnormalities in RV morphology and function on echocardiography [77, 78]. However, in patients with preexisting lung disease, the size and mass of the PE affects the PVR and RV injury. In general, the degree of pulmonary vascular obstruction on CT pulmonary angiography is a poor predictor of outcome, although a central location of clots tends to worsen prognosis [79]. Cardiogenic shock occurs in 10 % of acute PE cases, with a mortality rate of up 52 % [80]. RV dysfunction is observed in about 40–60 % of normotensive patients with PE. While mortality in the latter patients is only 8–10 % [35, 81, 82], RV dysfunction persists in approximately 40 % of previously healthy patients, manifesting itself as an abnormal RV on echocardiogram and/or as persistent exercise intolerance [83, 84]. While it has traditionally been thought that acute PE increases the afterload by mechanically obstructing the pulmonary vasculature, it has now been established that the release of platelet- and endothelial-derived mediators (e.g., serotonin, endothelin-1, thromboxane-A2, prostaglandin F2α) exerts vasoconstrictor effects even in non-obstructed areas and further contributes to the increase in PAP [65, 85, 86]. Similarly, PE-associated intracardiac hemolysis (resulting from severe tricuspid regurgitation and turbulent flow across the pulmonary valve) contributes to vasoconstriction and an increase in the afterload via nitric oxide scavenging by free hemoglobin and via l-arginine depletion (the latter via release of erythrocyte arginase) [87–89]. In fact, plasma haptoglobin concentrations decrease as the severity of PE and the elevations in RVSP increase [87]. Other embolism syndromes associated with acute RV dysfunction include fat, amniotic fluid, cement, or tumor emboli [90–92].
Although any form of chronic PH can result in acute RV dysfunction, overt RVF is frequently encountered in patients with pulmonary arterial hypertension (PAH) or chronic thromboembolic pulmonary hypertension (CTEPH) [31, 93, 94]. In both syndromes, acute RVF can be the initial presentation of the disease, or present as acute-on-chronic RV failure. Acute PH and RVF are serious problems after cardiothoracic surgery. Mechanisms are multifactorial and include depression of preload and cardiac contractile strength in response to a systemic inflammatory response syndrome, as well as increased afterload from lung vascular microembolism, effects of mechanical ventilation, cardiac restrictive physiology, and the superimposed negative inotropy by drugs required for sedation or heart rate control [95–97]. Specific risk factors for the development of perioperative RVF include the use of cardiopulmonary bypass, RV ischemia-reperfusion injury, and placement of left ventricular assist devices (LVADs) [98, 99]. The etiology for RV dysfunction in the latter case has not been fully characterized; changes in geometry and contraction patterns after LVAD placement have been proposed (see below) [100–102]. Sickle cell crisis, specifically the acute chest syndrome (ACS), has also been characterized by marked elevation in tricuspid regurgitant jet velocity in as many as 60 % of patients [103]. Cor pulmonale has been described in as many as 13 % of ACS patients [103]. The mechanism underlying the development of PH and subsequent RVF in the setting of ACS is multifactorial and includes hyperhemolysis, HPV, pulmonary microvascular embolism and thrombosis, bone marrow emboli, and coronary hypoperfusion [103–105]. Importantly, ACS-associated PH and RV dysfunction are associated with cardiac biomarker elevation and a higher risk of death [105].
Hypotensive states, such as sepsis, shock, hemorrhage, or severe hypovolemia, compromise both RV filling as well as RV coronary perfusion [2]. Sepsis and shock have complex effects on RV function, as they—in addition to decreasing preload—can directly decrease RV contractility via negative inotropic effects of bacterial endotoxins and/or pro-inflammatory cytokines (e.g., TNF-alpha, IL-1beta, IL-6) [4, 106]. Furthermore, sepsis-induced endothelial dysfunction with pulmonary vasoconstriction and pulmonary microthrombi can significantly increase RV afterload [4, 107]. Lastly, treatments employed for sepsis and/or shock (MV, overzealous volume resuscitation) can negatively affect RV function [2, 8, 31].
Severe respiratory disease can cause significant RV dysfunction, as hypoxemia and hypercapnea may impair RV performance via vasoconstrictor effects, and potentially also via direct effects on the myocardium [2, 8, 31, 108, 109]. ARDS has been associated with increased transpulmonary gradients in 73 % of patients in the ARDSnet cohort [110], and acute cor pulmonale occurs in 25–50 % of ARDS patients, depending on the severity of the syndrome [21, 111, 112]. In addition to hypoxic/hypercarbic vasoconstriction, mechanisms of ARDS-induced RV dysfunction include pulmonary microthrombi, pro-inflammatory cytokine activation, and negative effects of MV (see below) [21, 111, 113, 114]. An increased alveolar dead space, resulting from either precapillary occlusion or globally reduced lung perfusion from RV failure, independently increases the risk of death from ARDS [115, 116]. A recent study identified infection-induced ARDS and airway pressures as independent predictors of ARDS-induced cor pulmonale [112]. Another study identified a PaCO2 ≥ 60 mmHg as a risk factor [113]. However, while ARDS-induced PH and cor pulmonale have been identified as risk factors for death, these results have not been uniformly confirmed [110, 112, 113]. Lastly, exacerbations of chronic obstructive airway diseases may be accompanied by air trapping; the letter may negatively impact pulmonary venous return to the RV (see also Chap. 18).
Mechanical ventilation may also adversely affect RV function, especially in the setting of preexisting chronic RV dysfunction, and if high pressures and/or high tidal volumes are employed. The dynamics of the effects of positive pressure ventilation on RV mechanics are complex and involve reduction in RV preload, increase in afterload (by converting West zone 3 to zone 1 or 2 conditions), and impaired LV filling [117–119]. Severe hypoxemia and/or hypercapnea are commonly encountered in patients requiring MV and likely contribute to RV dysfunction [113].
In LVAD recipients, both short- and long-term outcomes largely depend on RV performance after installation of the circulatory support device [98, 100, 120, 121]. Unfortunately, the development of RVF after LVAD implantation occurs in up to 30 % of cases [98, 122]. Whether this is the result of inducible RV dysfunction or simply “uncovering” a preexisting RV dysfunction remains controversial. Some earlier experimental models suggest that during LVAD support, despite initial improvements in reduction in RV afterload, RV contractility is significantly impaired due to geometrical changes in the LV and subsequent leftward shift of the IVS [100, 101]. However, a recent study of 130 patients with chronic LVF who underwent placement of continuous-flow LVAD actually showed significant improvements in RV performance as determined by an increase in RVEF, RV stroke work index, tricuspid annular plane systolic excursion (TAPSE), and reduction in RV end-diastolic dimensions [120].
Because of these conflicting findings, there has been a strong interest in predicting which patients are at risk of developing progressive RVF after initiation of LVAD support. It is known that patients requiring late biventricular VAD (BiVAD) support have poor outcomes [123–126]. For example, among LVAD recipients who eventually required additional RVAD support, only 35.7 % were bridged to transplantation when compared to 89.9 % of those with LVAD therapy alone [124]. Similar outcomes (40 % survival at time of transplant in those needing BiVAD support vs. 100 % in the LVAD group) were reported in another study [126]. Early installment of BiVAD therapy in high-risk patients significantly improves survival compared to patients with delayed insertion of RVAD support (51 % vs. 29 %) [123]. Patients requiring aggressive inotropic support or intra-aortic balloon counterpulsation devices prior to installation of VAD therapy are among the highest risk patients for developing deterioration of RV function post-VAD placement [99]. Obesity, severe hemodynamic alterations such as elevated PVR, and use of LVAD as destination therapy were also found to be strongly associated with development of RVF [99].
Intrinsic cardiac disease, including acute RV myocardial infarction (RVMI), acute valvulopathies (e.g., mitral or tricuspid regurgitation), viral myocarditis, septic cardiomyopathy, and pericardial disease (e.g., cardiac tamponade) can be complicated by acute RVF [8, 127, 128]. RVMI has been traditionally associated with hypotension, distended jugular veins, and clear lung fields in patients suffering from an acute MI [129]; however, hemodynamically significant RVMI presenting with hypotension actually occurs less than 10 % of the time [129]. Typically the RCA is the culprit vessel, with more extensive RV myocardial necrosis associated with the proximity of the occlusion [130]. The prognosis of RVMI largely depends on presence of complications, such as ventricular arrhythmias or a high-grade atrioventricular block, underlying RV dysfunction, and the success of angioplasty to restore coronary reperfusion [8, 130–132].
An interesting recently identified cause of acute RV dysfunction and sudden cardiac death is the syndrome of arrhythmogenic RV dysfunction in endurance athletes [133] (see Chap. 16). Similarly, it has recently been demonstrated that acute, high-intensity exercise can result in acute dysfunction and cardiomyocyte damage of the RV, but not the LV [134] (see Chap. 15). A decrease in RV function can also be seen after brain death and—in case of heart transplantation—may contribute to early postoperative RV failure in the recipient [135, 136].
Lastly, patients with comorbidities associated with chronic RV dysfunction (e.g., obstructive sleep apnea, chronic lung disease, chronic LV dysfunction, PAH) are at risk for acute decompensation of RV function either due to progression of their underlying disease, or as a consequence of an additional insult to the RV (Table 9.2).
Table 9.2
Common causes of acute-on-chronic right ventricular failure
New onset atrial/ventricular arrhythmias |
Acute pulmonary embolism |
Acute myocardial ischemia/infarction |
Progression of underlying disease |
• PAH |
• Chronic lung disease |
• CHF |
Acute hypoxemia and/or hypercarbia |
• COPD/IPF exacerbation |
• Pneumonia |
• ARDS |
• High altitude |
Untreated or worsening sleep disorders |
• Obstructive sleep apnea |
• Obesity hypoventilation syndrome |
• Nocturnal hypoxemia |
New or worsening anemia |
Infection |
• Sepsis |
• Catheter-related bloodstream infection in PAH patients on chronic prostacyclin therapy |
Iatrogenic |
• Mechanical ventilation |
• Over-diuresis |
• Aggressive fluid resuscitation |
Medications |
• NSAIDs |
• Nondihydropyridine calcium channel blockers |
• Nitrates |
Behavioral |
• Medication nonadherence |
• Dietary nonadherence |
Diagnosis and Risk Stratification
The management of acute RVF begins with recognizing the key signs and symptoms to make the initial diagnosis. Currently available tools include history and physical examination, electrocardiography, biomarkers, imaging studies, noninvasive hemodynamic monitoring devices, and invasive cardiopulmonary hemodynamic monitoring. Importantly, the same tools used for diagnosing RV dysfunction have also been applied to predicting patient outcomes, and are being used for both risk stratification and management of acute RVF (Table 9.3).
Table 9.3
Overview of commonly used tools for diagnostic testing and risk stratification in acute right ventricular failure
Serum biomarkers | |
B-type natriuretic peptide (BNP), NT-proBNP | • NT-proBNP >1,400 pg/mL association with increased mortality in RVF in PAH [152] |
• BNP >180 pg/mL associated with significant increase risk of death in PAH [153] | |
• BNP >90–100 pg/mL associated with increased mortality and adverse clinical events in RVF in acute PE [149] | |
• BNP >168 pg/mL indicates severe RV dysfunction in patients with CTEPH [151] | |
Cardiac troponins | • Independent predictor of death in acute PE; elevated levels associated with increase in short-term mortality, death secondary to PE, and adverse outcomes [157] |
• Also predictor of mortality in PAH [152] | |
C-reactive protein (CRP) | |
• CRP >5 mg/dL is associated with decreased survival and event-free survival in patients with PAH [302] | |
• Correlates with severity of PAH [302] | |
Sodium (Na), glomerular filtration rate (GFR) | • Na <135 mmol/L associated with increased 30-day mortality and an independent predictor for hospital readmission in acute PE [161] |
• Na <136 mmol/L predicts frank RVF and increase risk of death in PAH patients [160] | |
• GFR <45 mL/min per 1.73 m2 associated with increased likelihood of death or urgent transplantation in acute PAH-induced RVF [159] | |
Echocardiographic parameters | |
Pericardial effusion | • Echocardiographic findings which predict poor outcomes in PAH [153] |
RA, RV enlargement | |
Septal displacement or “bowing” | • RV/LV diameter >0.9 predicts increased mortality in acute PE [143] |
RV hypokinesis | • Bowing of the IVS associated with short-term mortality [186] |
Severe tricuspid regurgitation | |
RV diameter/LV diameter ratio | |
Tricuspid annular plane systolic excursion (TAPSE) | • TAPSE ≤1.5 cm predicts increased mortality, re-infarction rates, and hospitalization at 2-year follow-up in setting of RVMI [188] |
• TAPSE ≤1.8 cm indicates more severe RV dysfunction and worse overall prognosis [189] | |
• TAPSE ≤1.8 cm in setting of acute PE predicts worse 1-year survival [190] | |
Presence of RV notching | • Mid-systolic notching predicts increased PVR and more severe RV dysfunction in PAH [194] |
Tissue Doppler, Tei index, RV myocardial performance index (RVMPI) | • Newer indices reliable for assessing RVF; less preload dependent [25] |
Hemodynamic parameters | |
Right atrial pressure (RAP) | • RAP >15 mmHg indication for transplant referral for PAH [200] |
• RAP >20 mmHg associated with significant increased risk of death in PAH [153] | |
• RAP >20 mmHg contraindication for balloon atrial septostomy [200] | |
RV stroke work index (RVSWI) | • RVSWI ≤0.25 mmHg × mL/m2 strong predictor of RVF after LVAD placement [123] |
History, Patient Factors, and Physical Examination: As in chronic RVF, signs and symptoms of acute RVF tend to be nonspecific and late. While patients typically present with new onset signs and symptoms of RVF, in cases of acute-on-chronic RVF patients likely have preexisting symptoms and physical examination findings. As in chronic RVF, symptoms of chest pain, lightheadedness, and syncope, as well as findings such as hypotension, cyanosis, and cool extremities represent severe and advanced RV dysfunction [137–139].
Over recent years, the patterns of clinical presentations of acute RVF have been included in the risk stratification and management strategies of these patients. For example, syncope in patients with acute PE or advanced PAH strongly correlates with severe RV dysfunction and is associated with increased overall mortality [19, 139, 140]. Signs of hemodynamic instability (i.e., hypotension and tachycardia) also are strong predictors of poor outcomes in patients with PAH [141] or acute PE [19, 35, 142]. Similarly, an altered mental status predicts poor outcomes in acute PE and is included as a component of a recently published severity index [143]. Age and comorbidities influence the prognosis in acute PE [35, 81, 144], and have been included in both the Geneva and pulmonary embolism severity index (PESI) scores [145, 146]. Male sex is a risk factor for death in PAH [147]. Connective tissue disease is a risk factor for death in PAH patients admitted with RVF [141]. Knowledge of these prognostic findings is fundamental in early risk stratification and allows the opportunity for initiation of appropriate therapies.
Invasive and Noninvasive Studies for Diagnosis and Risk Stratification
Though direct hemodynamic assessment via right heart catheterization is critical for the diagnosis, classification, and management of acute RVF, this is frequently complemented by important noninvasive tests including biomarkers, echocardiography, and radiographic imaging.
Biomarkers: Though nonspecific and potentially confounded by other disease states (e.g., LV disease, renal failure, obesity), increased levels of biomarker can allow assessment of the RV dysfunction. Used in conjunction with the clinical presentation, biomarkers have also been invaluable in assessing disease severity and guiding therapy. A major advantage of the use of serum biomarkers is their high negative predictive value (NPV); biomarkers in the normal range essentially rule out RVF (with obesity being a potential exception to this rule).
BNP and NT–proBNP are well established in both diagnosis and management in LVF [148]; however their role in RV disease is increasingly being recognized. Regardless of the cause of RV dysfunction, in the absence of LV or renal disease, elevated natriuretic peptide concentrations predict the severity of RV dysfunction observed on echocardiography [19, 149–152]. In patients with PAH or PE, elevated NT-proBNP has a strong prognostic value predicting death from decompensated RV function. For example, in PAH, proBNP concentrations >1,400 pg/mL have a sensitivity and specificity of 88 % and 53 %, respectively, and a NPV of 91 % for predicting fatal outcomes [152]. Similarly, BNP levels >180 pg/mL have been associated with poor outcomes [153]. Increased BNP also predicts adverse clinical events and increased mortality in patients with acute RVF secondary to acute PE [19, 149]. Recent meta-analyses identified elevated BNP levels as significantly associated with increased short-term all-cause mortality, death resulting from PE, and serious adverse events [149, 154–156]. For example, in a meta-analysis by Sanchez et al. [156], the odds ratios for short-term mortality for BNP or N-terminal pro-BNP elevations in patients with submassive PE were 9.51 and 5.74, respectively. The recent AHA Statement on Massive and Submassive Pulmonary Embolism considers BNP >100 pg/mL or NT-pro-BNP >900 pg/mL as strong markers of moderate-to-severe RV strain [9].
Cardiac troponins indicate cardiomyocyte death and as such, represent a relatively late finding in the progression of RV dysfunction toward frank RVF. An abnormally elevated troponin I or T (generally defined as higher than the 99th percentile for the coefficient of variability <10 %), has a strong positive predictive value for poor global RV contractile function and death, in normotensive patients [19, 142, 157, 158]. In submassive PE, troponin elevations are associated with an odds ratio for mortality of 5.9 [19]. Interestingly, in acute PE, the combination of RV dysfunction as judged by echocardiography and elevated troponin seems to be more predictive of inhospital death or clinical deterioration, than either parameter alone [142].
Other biomarkers have been shown to be important prognostic markers in PAH patients with acute RVF admitted to the ICU [17]. These include elevated liver function tests, increased creatinine, and elevated C-reactive protein. In particular, an increase in serum creatinine is associated with poor outcomes [17, 159]. Hyponatremia, a sentinel marker of increased neurohormonal activation, is associated with poor outcomes in both RVF secondary to acute PE as well as patients with acute RVF from decompensated PAH [17, 141, 159–161].
Emerging biomarkers: Heart-type fatty acid-binding protein (H-FABP) has recently been suggested as a marker of RV stress in normotensive patients with acute PE. H-FABP levels ≥6 ng/mL were associated with a 36.6-fold increase in death or complication risk [162]. Elevated levels predicted worse clinical outcomes even in the setting of a normal echocardiogram at the time of PE diagnosis [163]. However, in patients with an abnormal echocardiogram, high levels of H-FABP seemed to be even more predictive of a worse clinical outcome [163]. Growth differentiation factor-15 (GDF-15) is a member of the transforming growth factor-β cytokine family that is produced by pressure-overloaded or ischemic myocardial tissue [164, 165]. GDF-15 > 1,200 ng/L is strongly associated with hemodynamic abnormalities and increased risk of death or lung transplantation in RVF secondary to PAH [166]. In acute PE, concentrations >4,600 ng/L have a prognostic sensitivity of 71 %, specificity of 90 %, and NPV of 95 % for complicated 30-day clinical outcomes, and also predict increased long-term mortality [167].
Electrocardiography: The electrocardiogram (ECG) may suggest an underlying RV etiology if it demonstrates right axis deviation, P pulmonale, RV hypertrophy, right-sided conduction delay, or RV strain pattern (e.g., S1Q3 pattern or T-wave inversion in the precordial leads; Fig. 9.4d). ECG patterns have also been demonstrated to correlate with disease severity. For example, atrial arrhythmias, new right bundle branch block (complete or incomplete), Qr pattern in V1, S1Q3T3, negative T-waves in V1 through V4, and ST-segment shift over V1 through V4 have been associated with worse outcomes, including inhospital deaths, in patients presenting with acute PE [9, 19, 168]. However, while specific, the use of ECG in acute RV dysfunction is limited by a lack of sensitivity [2]. In the setting of acute PE, a numeric score has been derived and validated that predicts the PAP and degree of perfusion defects on the lung scan [169–171].


Fig. 9.4
Noninvasive diagnostic methods for acute right ventricular failure (RVF). (a, b) Echocardiographic images from a 40-year-old female with acute RVF from scleroderma-associated PAH demonstrating incomplete tricuspid valve coaptation (white arrow) during systole (a) and compression of the LV during diastole (b; known as the “D-sign” [white arrowhead]), both consistent with severe RV pressure overload. Note presence of severe right atrial (RA) enlargement and left atrial (LA) underfilling. (c) Color tissue Doppler sampling of the tricuspid annulus in four-chamber view of the same patient reveals significant reduction in RV systolic velocity suggestive of severe RV systolic dysfunction. (d) EKG from the same patient is demonstrating right axis deviation, peaked p-waves in lead II, and significant T-wave inversions in precordial leads suggestive of severe right atrial enlargement and RV strain, respectively. Precordial leads also demonstrate evidence of RV hypertrophy. (e, f) Contrasted chest computer tomography (CT) images of a 46-year-old male presenting with acute massive pulmonary embolism. Note significant RA enlargement and RV dilatation with reversal of appropriate RV to LV size ratio (e; RV/LV ratio >1) and severe hepatojugular reflux of intravenous contrast into inferior vena cava and hepatic veins (asterisk in f). The latter finding has been associated with turbulent flow in the RV and subsequent hemolysis [87, 172]. LA left atrium, LV left ventricle, PA pulmonary artery, RA right atrium, RV right ventricle
Radiographic Studies: Plain chest radiography lacks sensitivity, but may have some utility in determining the etiology of acute RVF. For example, cardiomegaly, pleural effusions, and pulmonary edema can be suggestive of LVF as the cause of RVF. Pulmonary infiltrates may suggest a primary lung pathology. Clear lung fields with prominent pulmonary vasculature on the other hand may suggest decompensated PAH as the underlying etiology. Findings of RV dysfunction on chest computed tomography (CT), such as right atrial (RA) and RV dilatation, leftward shift of the IVS, or reflux of intravenous contrast into the hepatic veins, are often late findings of disease and may suggest increased mortality [2, 172] (Fig. 9.4e + f ). Since chest CT is often performed for other reasons, such findings are not infrequently picked up incidentally. In acute PE, CT evidence of RV dilation (RV/LV diameter >0.9 in a four-chamber view [9]) predicts worsened findings on echocardiography, but prospective studies have failed to find an independent association with adverse short-term events, including death in the hospital, at 30 days, or at 3 months [79, 173]. Chest CT with intravenous contrast is frequently obtained during the initial evaluation of acute PE. However, for the evaluation of CTEPH as the cause of acute RV dysfunction, chest CT with intravenous contrast lacks sensitivity, and ventilation/perfusion scanning appears to be more sensitive [174]. Chest CT may also help to identify pulmonary parenchymal or cardiovascular causes of RVF. Cardiac magnetic resonance imaging (cMRI) is considered the gold standard for the noninvasive evaluation of RV and LV function and structure [25, 175]. However, due to practical reasons, its use for the diagnosis and management of acute RVF is limited, as these patients are frequently not stable.
Echocardiography: Echocardiography is an invaluable tool for both diagnosis and management of acute RV dysfunction [2]. Traditional parameters of RV dysfunction such as RA enlargement, RV dilatation, RV hypokinesis, leftward shift of the IVS, significant tricuspid regurgitation, and dilatation of the inferior vena cava with lack of respiratory variation predict adverse outcomes in patients with acute PE or decompensated PAH (Fig. 9.4a–c) [19, 175–178]. The estimation of RVSP via the modified Bernoulli equation is fundamental in screening patients for elevated PAPs. However, RVSP, as estimated by echocardiography, may over- or underestimate the true systolic PAP in PH patients by >10 mmHg in almost 50 % of cases, especially when patients have intrinsic lung disease [179, 180]. To further complicate the use of estimated RVSP, in the setting of acute RVF, a decrease often reflects worsening RV dysfunction and a low CO [181]. Therefore, in decompensated RV disease, RVSP values should be considered a screening step and additional markers of RV performance are required. Despite these limitations, in acute PE, very high RVSP (e.g., >50 mmHg) at diagnosis predicts a high probability of the patient experiencing persistently elevated PAPs with standard anticoagulation [182–185].
In patients with acute PE, the increased RV/LV diameter ratio has been identified as an independent predictor of mortality [143]. While this ratio was not identified as a predictor of death in acute PE patients previously, that study identified bowing of the IVS as a predictor of short-term mortality [186]. In patients with acute myocardial infarction, presence of RV free wall hypokinesis, decreased TAPSE, and reduced lateral tricuspid annular velocity on tissue Doppler imaging (TDI) may be suggestive of RV involvement [3, 175, 187]. A TAPSE ≤1.5 cm and presence of RV strain independently predict mortality, re-infarction rates, and hospitalization at 2-year follow-up in patients with acute MI [188]. In patients with PAH, TAPSE <1.8 cm reflects more severe RV dysfunction and a poorer prognosis [189]. The TAPSE may also indicate RV dysfunction in patients with acute PE, as lower values indicate elevated RV pressures [190]. Newer indices such as tissue Doppler, Tei index, and RV myocardial performance index (RVMPI) are being evaluated in patients with PH and/or acute PE [191–193]. A detailed overview of prognostic echocardiographic parameters is provided in Table 9.3.
Echocardiography also offers clues that help uncover the etiology behind decompensated RVF. For example, injection of agitated saline allows for detection of intracardiac shunts as a possible cause or consequence of RVF. Findings of left atrial enlargement and LV hypertrophy are more suggestive of LV pathology as the cause of RV failure. Notching of the Doppler signal in the RV outflow tract reflects significant pulmonary vascular remodeling and is suggestive of PAH [194]. Once thought to be a specific finding for acute PE, the McConnell sign refers to a distinct pattern of RV mid-free wall hypokinesis with preserved contraction of the apical segments; however, this sign has been increasingly identified in other forms of RVF [195–197]. Transesophageal echocardiography (TEE) has been proposed as a superior method when compared to transthoracic echocardiography for detecting RV dysfunction in ARDS [113], but further studies are needed before such a strategy can be recommended.
Invasive Hemodynamic Assessment: Right heart catheterization remains the gold standard for diagnosing and thoroughly characterizing acute RVF and determining its etiology. It also provides significant insight into both disease severity and prognosis [198]. While right heart catheterization in general is a very safe procedure [199], PA catheters (PACs) may be more difficult to float in patients with RVF, and their placement is associated with a higher rate of complications [5], a potential problem in patients with already compromised cardiopulmonary hemodynamics. PAC placement-associated tachyarrhythmias may be particularly devastating. Thus, the procedure should ideally be performed in the cardiac catheterization lab, but if the patient is too unstable to be transported, PA catheterization can be performed in the ICU. In addition to directly measuring RA pressure, PAP, and pulmonary artery wedge pressure (PAWP), right heart catheterization allows the measurement and/or calculation of right atrial pressure (RAP), CO and cardiac index (CI), mixed SvO2, PVR, and RV stroke work index [74, 200–202]. Measurement of these parameters in the setting of acute RVF assists with guiding the therapy (inotropic agents, pulmonary vasodilators, and diuretics). Assessment of oxygen saturation in sequential right heart chambers (e.g., RA and RV) help determine the presence of a significant left-to-right shunt as a possible cause of RVF. Again as a reduction in PAP may reflect decreasing RVEF and worsening RVF [181].
While determination of Pcwp as a surrogate for LV end-diastolic pressure (LVEDP) is critical in the evaluation of RV dysfunction, one needs to keep in mind that the Pcwp can be poorly calibrated to LVEDP. Even in regular outpatient populations, the Pcwp over- or underestimates the LVEDP in a large percentage of patients [203, 204]. Since the presence of tachyarrhythmias, respiratory excursions, and MV may negate the assumption of a static blood column between the catheter tip and LV, the agreement between Pcwp and LVEDP may be even less robust in acute RVF patients. For example, accurate measurement of Pcwp in patients in the ICU is often complicated by the presence of intrinsic lung disease, incorrect catheter placement, and MV (e.g., presence of positive end-expiratory pressure [PEEP]) [205, 206]. Specifically, in the presence of higher levels of PEEP, Pcwp can be falsely elevated. A more accurate approximation of Pcwp in a patient on MV and high level of PEEP can be made by subtracting about one-half the PEEP level from the Pcwp. Acidosis, hypoxemia, and administration of vasoactive drugs may also influence the accuracy of Pcwp measurements [206]. Depending on the stability of the patient, performance of left heart catheterization may be desirable, as it allows a direct measurement of the LVEDP and assessment of coronary artery disease.
Novel methods for assessment of afterload and RV–PA coupling include the use of impedance catheters and the measurement of pressure–volume loops in the RV [36]. While these techniques are highly promising and while they may provide critical information their use is currently limited to research.
Noninvasive Hemodynamic Monitoring: Recently, there has been an increasing use of noninvasive hemodynamic monitoring techniques during the management of hemodynamically unstable patients (e.g., measurement of variations in pulse pressure, systolic blood pressure, or stroke volume) [207–209]. In studies evaluating mechanically ventilated hypotensive patients with an absence of arrhythmias, these techniques appear to be promising. However, since patients with RVF frequently exhibit increased respiratory efforts and/or tachyarrhythmias, extrapolation of these data to patients with RVF is problematic. Passive leg raising may better predict fluid responsiveness in patients with arrhythmias and spontaneous respiration [210]. A recent study evaluated a bioreactance-based noninvasive CO monitoring method, and found this to be precise and reliable in a stable PH population [211]. However, the validation in patients with acute RVF is needed.
Management of Acute RVF
The key principle in the management of acute RVF focuses on determination and treatment of the underlying etiology. Specific therapies are usually complemented by supportive strategies focused on improving RV function via volume optimization, enhancing contractility, and reducing afterload (Fig. 9.5). These goals are achieved through a combination of appropriate volume management, vasopressors and/or inotropic agents, pulmonary vasodilators, and interventional or surgical strategies [2]. Unfortunately, no RV-specific therapies exist, and most of the interventions aimed at improving RV performance and/or PA vasomotor tone concomitantly affect the systemic vasculature and/or the LV. In addition, studies specifically evaluating patients with RVF are few, limited by small patient numbers and they are usually not prospective. The management of patients with acute RVF should always incorporate general preventative measures, supportive care, and specific interventions aimed at the underlying cause.


Fig. 9.5
Treatment of acute RV failure. Treatment of the failing RV focuses on attenuation of pathophysiologic alterations in preload, contractility, and afterload by a combination of general methods and specific therapies directed against the underlying, as well as pharmacological and interventional approaches directed against the failing RV. Recent studies suggest potential role for epoprostenol and PDE-5 inhibitors to improve RV contractility, the later through concomitant PDE-3 inhibition. Use of milrinone, dobutamine, and levosimendan also have concurrent benefits in afterload reduction. Importantly, management of acute RVF depends on general supportive care including oxygen therapy, volume optimization, and correcting anemia, if present. Specific therapies, such as thrombolytic therapy for acute pulmonary embolism, early percutaneous coronary intervention for acute RV myocardial infarction, or antibiotic therapy and volume resuscitation for patients with sepsis are indicated in the appropriate clinical setting for additional management of RVF. BiVAD biventricular assist device, NO nitric oxide, RVAD right ventricular assist device
General Management and Supportive Care
General measures: The initial focus in any patient with acute RVF, irrespective of the underlying etiology, is early evaluation of any underlying reversible factors that are either primarily responsible for or contributing to the deterioration of RV function [2, 17, 31, 212, 213]. Infections are one of the strongest predictors of mortality in patients with RVF secondary to PAH [17, 31]. Similarly, infection has been identified as a risk factor for ARDS-induced cor pulmonale [112]. Thus, the importance of preventative measures, aggressive monitoring for infections, and early use of appropriate antibiotics and source control measures cannot be overemphasized. Anemia can be deleterious to RV function, as it may result in decreased oxygen carrying capacity and reduced oxygen delivery to the failing RV, while simultaneously increasing the oxygen demand as a consequence of anemia-induced tachycardia. The optimal hemoglobin (Hb) level for patients with acute RVF is not well defined [31]. While typical ICU patients may benefit from a conservative transfusion strategy [214], this specific patient population may require higher hemoglobin levels in order to maintain appropriate oxygen delivery [215]. Monitoring serum lactate levels and central or mixed venous oxygen saturation (ScvO2 or SvO2) may assist in guiding decisions regarding the need for transfusions [10, 216].
Management of supraventricular arrhythmias: Supraventricular arrhythmias can be highly detrimental to RV performance, thus reflecting the importance of atrial contraction in maintaining RV diastolic filling and RV stroke volume [25]; a recent study in a PAH and CTEPH cohort with atrial flutter or fibrillation demonstrated benefit from a rhythm control strategy [55]. In particular, both atrial flutter and fibrillation were strongly associated with clinical worsening, RVF, and death [55]. Whether these data can be extrapolated to other populations with RV dysfunction is unknown; however, immediate cardioversion in patients with significant RV dysfunction should be considered [5, 31].
Volume management is fundamental in acute RVF. Typically, patients with RVF present with volume overload and require sodium restriction and diuresis; thus the use of diuretics or renal replacement therapy is frequently required during the initial management [31]. Continuous infusion of diuretics may be preferable over bolus dosing, and the combination of a loop diuretic with a thiazide or thiazide-like diuretic (e.g., metolazone) may confer additional benefits [217, 218]. RVF is characterized by significant neurohormonal activation [17, 160], and emerging data indicate that inhibition of the renin–angiotensin–aldosterone system may provide significant clinical benefits [219–221]. While initial data suggested a role for ultrafiltration for patients with LVF and cardiorenal syndrome, the recent CARRESS–HF trial found that ultrafiltration was inferior to pharmacological therapy in this population, and resulted in a significant increase in serum creatinine and serious adverse events [222]. The routine use of this strategy for acute RVF can thus not be recommended. Over-diuresis, however, may be detrimental to RV function, leading to electrolyte imbalances, reduced CO, pre-renal azotemia, and systemic hypotension. A commonly used strategy is to diurese until BUN or creatinine is starting to rise [223].
On the other hand, volume administration to an already overloaded RV will further impair CO through ventricular interdependence and subsequent underfilling of the LV [26]. In hypotensive patients with suspected hypovolemia, careful fluid boluses of 500 mL are recommended, but should be discontinued if no improvement in blood pressure or CO occurs after 1,000 mL [2, 5]. Use of clinical history, physical exam, bedside echocardiography, and serum biomarkers cannot be overemphasized in guiding volume management for RV dysfunction and failure. Frequently, invasive monitoring of hemodynamics is required.
The diabolical effect of preload reduction: The failing RV requires an adequate preload to sustain forward flow. However, many patients with severe afterload-induced RV dysfunction will have chest pain suggestive of angina. Usual care often prescribes the use of nitroglycerine in effort to improve coronary perfusion. Regrettably, nitrates have the opposite effect. Although clinical studies are lacking, in our experience, the use of nitrates in afterload-induced RV failure can produce a deleterious reduction in arterial blood pressure, especially in patients with acute PE. A similar phenomenon has been described for patients with acute RVMI.
Ventilation and oxygenation strategies: Maintaining oxygen saturation >90–92 % is crucial to avoid HPV. Similarly, excessive hypercapnia and acidosis should be avoided [5, 109]. On the other hand, due to its potential for reducing CO, causing hypotension and hemodynamic collapse, MV—if at all possible—should be avoided [117–119]. If MV is unavoidable, the lowest possible PEEP and tidal volume (VT) required to provide adequate oxygenation and ventilation, respectively, should be applied, and use of an ARDSnet strategy has been recommended [2, 5, 31, 224, 225]. However, a small VT may lead to hypercapnia with subsequent pulmonary vasoconstriction and increased afterload. While this may be overcome by increasing the respiratory rate, that maneuver may result in excessive auto-PEEP, air trapping, and preload reduction. Prone position ventilation may unload the RV [21, 226, 227], and a decrease in RV dysfunction has been proposed as one of the reasons for the positive results of a recent proning trial [226, 227]. However, this hypothesis will need a prospective evaluation. The complexities reviewed here emphasize why MV in acute RVF is associated with high morbidity and mortality [97, 141, 228]. For this reason, we recommend avoiding MV altogether by using extracorporeal membrane oxygenation (ECMO) in awake patients with RVF (see below) [31, 228, 229].
Specific therapies: A key principle in the management of acute RVF focuses on determination and treatment of the underlying etiology. Sepsis is treated with antibiotics, source control measures, volume replacement and, if needed, hemodynamic support [11]. ARDS treatment requires appropriate MV strategies (see above). PH crisis and RVF in PAH and in the postoperative setting are treated with the pulmonary vasodilators and inotropes (reviewed below). Patients with sickle cell crisis and ACS require blood transfusions and supportive care [230]. Patients with RVMI benefit from immediate administration of both anti-platelet and anticoagulation therapy in conjunction with early percutaneous coronary intervention (PCI) [8, 129, 132, 231]. Successful revascularization with normalization of blood flow within the RCA results in prompt recovery of RV function and decreased inhospital mortality [132]. LVF is treated with inotropes, afterload reduction and volume management strategies [73]. Mechanical support (intraaortic balloon counterpulsation or LVAD therapy) is needed in severe cases. Treatment of RVF following LVAD requires supportive care, volume optimization, and inotropic support with or without use of pulmonary vasodilators [98]. Patients unresponsive to such medical therapies may require installment of an RVAD for additional support. Valvular heart disease is treated with percutaneous or surgical interventions.
Unless contraindications exist, acute PE is treated with anticoagulation. In patients with massive PE (systolic BP <90 mmHg for at least 15 min or requiring inotropic support not due to a cause other than a PE), thrombolytic therapy is a reasonable decision in the setting of acceptable risk of bleeding [9]. However, in the setting of submassive PE, defined as the presence of PE without systemic hypotension but with evidence of either RV dysfunction or myocardial necrosis (see definition section) [9], treatment with thrombolytic therapy remains currently controversial.
Meta-analyses of randomized controlled trials have consistently failed to find an improvement in mortality for patients with submassive pulmonary embolism [232]. However, considerable evidence suggests that fibrinolytics can improve patient outcomes related to RV dysfunction at two timepoints: short term (inhospital) and longer term (3–6 month follow-up). For example, an earlier randomized trial suggested that in selected patients with echocardiographic evidence of RV dysfunction and a low risk of bleeding, early treatment with alteplase plus heparin (as compared with conventional anticoagulation therapy) reduced the need for emergency therapeutic measures during the hospital stay [233]. A large, multinational, randomized trial to determine whether normotensive patients with RV dysfunction (detected on echocardiogram or CT scan), and evidence of myocardial injury (indicated by a positive troponin) may benefit from early thrombolytic treatment (PEITHO; ClinicalTrials.gov number NCT00639743) has completed enrollment. Preliminary results of this large randomized controlled trial of fibrinolysis for PE demonstrated a significant reduction in the rate of inhospital deterioration (defined by the composite endpoint of shock, hypotension or death) [234]. Four independent studies, including one observational study and two randomized controlled trials have demonstrated lower rates of echocardiographically defined PH and RV dysfunction with fibrinolysis [183, 184, 235, 236]. A recent randomized trial found improved functional capacity and quality of life measures with fibrinolysis treatment after submassive PE [237]. Moreover, in the aggregate of randomized controlled trials of fibrinolysis that performed right heart catheterization have demonstrated a more complete and significant reduction in mean PAP compared with placebo (Fig. 9.6a + b). Taken together, these studies suggest that treatment of submassive PE with fibrinolytics may reduce inhospital deterioration and the development of pulmonary hypertension, and may improve functional capacity and quality of life.
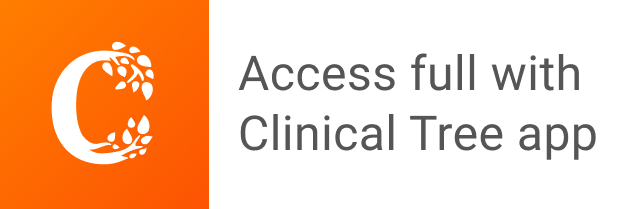