(1)
Kantonsspital Aarau, Medizinische Universitätsklinik, Aarau, Switzerland
Keywords
Heterometric adaptionHomeometric adaptionDiastolic ventricular interaction (interdependence)RV end-diastolic pressure (RVEDP)RV end-diastolic volume (RVEDV)Pulmonary vascular resistance (PVR)RV afterloadSeries-effectPulmonary hypertensionPulmonary vasculopathyPulmonary pressurePulmonary embolismRV contractilityRV enlargement/dilationMechanical ventilationSystemic congestionVasopressorsInotropic drugsSelective (pulmonary) vasodilators4.1 Definitions
Right ventricular failure (RV-F) is a complex, heterogeneous clinical syndrome, characterized by dyspnea—fatigue complaints and normally systemic congestion, which “can result from any structural or functional cardiovascular disorder that impairs the ability of the RV to fill or to eject blood” [1–3].
Thus, and in analogy to the definition of left ventricular (heart) failure, RV-F may be defined as:
“Inability of the RV to generate adequate forward flow with normal central venous pressure” [4].
A definition which has been endorsed by the fifth World Symposium on Pulmonary Hypertension in 2013 is set somewhat broader, but contains the same basic and essential pathological elements of heart failure, altered RV properties and performance, and the presence of increased filling pressures [5, 6]: “RV failure is a dyspnea fatigue syndrome with eventual systemic venous congestion, caused by the inability of the right ventricle to maintain flow output in response to metabolic demand without heterometric adaption, and consequent increase in right ventricular filling pressures” [7].
This definition outlines a wide range of clinical scenarios ranging from clinically a- or oligo-symptomatic and compensated conditions even under stress, which may be referred to as RV-D, however functional compensation is largely achieved by RV hypertrophy and in any way at the cost of elevated filling pressures, and ending with clinically overt malady with low output states and imminent circulatory collapse [3].
As such, right ventricular dysfunction (RV-D) is referred to as “abnormalities of RV-filling or RV-contraction without signs and symptoms of heart failure” [1].
4.2 Epidemiology and Aetiology
Right heart dysfunction/failure has a quite remarkable incidence, affecting approximately 5% of the US population [8] with the outcome largely depending on the underlying cause [9]. Three to nine percent of all admissions with acute heart failure syndromes (AHFS) are related predominantly to RV-F, with an in-hospital mortality rate being as high as 5–17% [10–13].
A wide variety of reasons altering right ventricular loading conditions, as occurring in case of pulmonary hypertension, or primarily diminished RV myocardial contractility as found in ischemia, cardiomyopathy, and arrhythmias, may lead to and provoke RV-D/RV-F [1, 2, 14].
Pulmonary hypertension (PH) actually is the most frequent pathogenetic feature causally involved in and contributing to RV-F genesis [14, 15]. Increases in pulmonary pressures, mostly due to and associated with an elevated pulmonary vascular resistance as the predominant underlying alteration in any setting [16], precipitate an increase in the input impedance1 of the pulmonary artery and thereby on the RV-outflow tract, thus afterload the right ventricle [14, 19–23]. RV failure is the potential consequence of this increased RV afterload [14].
RV pressure overload is usually associated with and due to LV-dysfunction [24]. The most evident and determining implication of LV failure is indeed a rise in LV intra-cardiac filling pressure, implying elevated downstream pulmonary pressures [25], causing pulmonary venous hypertension [26]. A diseased left heart, LHD, is the most common cause of PH at all [27, 28], and accounts for 65–80% of all PH cases [15, 28, 29].
Further, acute RV-D/RV-F due to acute pulmonary hypertension is a common condition in the ITU setting (overview by [21, 30–33]):
- (a)
Acute respiratory failure per se leads to an increase in pulmonary vascular resistance [34] and to a change in pulmonary compliance inducing an increase in RV-afterload [35]. Hypoventilation of the alveoli, hypoxia and/or hypercapnia from respiratory insufficiency (type I and type II) cause an increase in pulmonary pressure and thus promote PH [36–38]. ARDS is frequently associated with PH due to an increase in pulmonary vascular resistance (PVR) [34]. Pulmonary vascular resistance (PVR) may be elevated by an increase in lung volume (emphysema) and by any decrease in functional residual capacity, and so lead to RV-D/F [29]. It is well established that acute respiratory failure leads to an increase in pulmonary vascular resistance, an increase in RV-afterload and reduced RV-function [39–41].
- (b)
Mechanical ventilation (positive pressure ventilation) compromises the pulmonary (micro) circulation through an increase in transpulmonary pressure causing an increase in the systolic load of the RV (↑ RV-afterload) [33, 42–44]. With progressively increasing tidal volumes the RV has to generate a higher and higher pressure to open the pulmonary valve and to eject blood into the pulmonary vasculature [42, 45]. PEEP induces a rise in the intrathoracic pressure [46–50] and, at the very least, higher levels of PEEP (>8–10 cm H2O) will substantially increase the RV-afterload [46, 51, 52]. Thus, a protective ventilatory approach keeping the plateau pressure within the airways below 27 cm H2O, adapting pCO2 to less than 8 kPa (60 mmHg) and PEEP-levels as low as possible is required [53].
- (c)
“Aggressive” volume loading, not being unusual in intensive care units, increases RV preload, and may in already stressed but until then compensated RV conditions precipitate acute RV-D or even RV-F [54, 55]—animal studies demonstrated that chronically volume overloaded right ventricles are compensated and show normal contractility, but decompensate in case they are faced with any additional burden like sepsis or mechanical respiratory support [56, 57].
Factors contributing to an increase in pulmonary vascular resistance are [58, 59]:
Lung parenchymal destruction,
Airway collapse,
Microthrombi in the pulmonary vessels,
Excessive pulmonary vasoconstriction,
Hypercapnia,
General and local release of pulmonary vasoconstricting mediators.
These features underlying cellular and molecular pathways are characterized by an imbalance between endogenous vasoconstrictors (in particular endothelin-1) and vasodilators (in particular nitric oxide and prostaglandins) produced and secreted by the pulmonary endothelium leading to an increase in pulmonary vascular resistance and an elevated RV outflow impedance [15, 60–62]. As such, haemostatic imbalances, secondary to pulmonary endothelial dysfunction and/or injury considerably contribute to the rise in PVR [63].
Failure of the right ventricle is often the final and crucial point in acute critical illness [9, 64]. This is not at least because acute right heart failure substantially influences the LV performance in these conditions [65, 66]. In cases where cardiopulmonary resuscitation is necessary patients with moderate or severe pulmonary hypertension are unlikely to survive [67].
Accordingly, acute/acutely decompensated left heart failure, acute respiratory failure conditions including mechanical ventilation, acute coronary syndromes causing myocardial ischemia, particular if involving the RV, sepsis and other severe infections, and acute pulmonary embolism represent the vast majority of maladies underlying acute RV-D/RV-F [68–70].
Table 4.1 lists a range of reasons causing RV-F (adapted from predominantly Harjola [2], and others [19, 71]:
Table 4.1
Causes of RV-F and differential aetiological and diagnostic considerations
• Acute respiratory failure [33] due to | |
• Pericardial disease (tamponade) | |
• Cardiomyopathies | • Valvulopathies |
• Arrhythmias | • Congential heart disease |
• Pulmonary hypertension due to hematological, e.g. sickle cell disease, infectious, e.g. HIV, and miscellaneous systemic and vascular diseases e.g. sarcoidosis |
4.3 Physiology and Pathophysiology
4.3.1 General Physiological and Pathophysiological Issues
The main functions the right heart has to comply with are to accommodate the entire venous return and to transmit the blood into the pulmonary circulation for gas exchange [89, 90], thereby maintaining low right atrial (RA) and pulmonary pressures and optimizing varying amounts of venous return [15, 17, 83]. In order to do so, the right ventricle function has to integrate preload, afterload, contractility, pericardial constraint, interaction with the left ventricle, and cardiac rhythm [1, 3, 91].
The pressure difference between the pressure in the periphery (systemic filling pressure) and the right atrium (central venous pressure) determines the amount of venous return and ranges usually between 7 and 10 mmHg at which the RA-P is normally 0 mmHg [92]. In healthy persons, only a very low isovolumetric contraction pressure is needed to be generated by the RV [93, 94] in order to eject blood into the low-resistance, high-compliance and low-impedance pulmonary vessel system [95–97]. Thus, in healthy individuals with notable physiological LA filling pressures and pulmonary vascular resistance, a negligible RV contractile contribution is required to allocate adequate CO [17]: Simply the negative pleural pressures physiologically produced by normal breathing promote blood flow through the pulmonary circulation [97].
Indeed, the anatomical conditions of the right ventricle (thin-walled, crescent shaped in a cross sectional view, but triangular in a side view [98], and particularly the direct geometry of the RV) allow not only to adapt to large increases in right ventricular filling volumes due to high venous return [99, 100], but also, despite often dramatic varying amounts of venous return, to definitely maintain a more or less constant CO [101, 102]. This crescent shape with a concave free wall and a convex septum [99] means that the RV has a markedly lower volume to surface ratio in comparison to the left ventricle and thus a much higher compliance [103]. However, these anatomical features and physiological properties of both the RV (high compliance, increasing not declining wall stress during systole [17, 104]) and the pulmonary vascular tree (low-resistance, high-compliance and low-impedance), predispose the RV to significant chamber dilatation in case of acute after-loading [19, 103, 105–107], and imply that the right chamber can very poorly (even worse than the LV [108])—see Fig. 4.1—adapt to acute increases in PA input impedance [16, 19, 20, 109]. As such, in case RV afterload acutely increases, the until then healthy RV is found to be unable to generate mean pulmonary artery pressures of more than 40 mmHg [110].


Fig. 4.1
Illustrates the high sensitivity to afterload of both ventricles. Any increase in afterload affects the systolic performance, indicated by stroke volume, of the right ventricle markedly stronger than the left ventricle. The reduction in stroke volume is considerably higher in RV compared to LV for any increase in ventricular load imposed. Adapted from Greyson CR. The right ventricle and pulmonary circulation: basic concepts. Rev Esp Cardiol. 2010;63: 81–95 [17] with permission
Acutely elevated pulmonary pressure is the most frequent cause of acute right heart failure [14, 27–29]. It is predominantly an increased pulmonary vascular resistance (PVR) which leads to PH in any setting [16]. PH generally results from increases in pulmonary vascular resistance, pulmonary blood flow, pulmonary venous pressure, or a combination of these features [6, 111, 112]. Vasoconstriction (e.g. hypoxic alveolar vasoconstriction via direct pressor effects or due to mediators), thrombosis, and vascular remodelling may all cause PH [113], and are generally associated with increases in PVR [3, 15, 27, 41, 60, 114–117]. An elevated PVR indicates functional and/or structural pulmonary vasculopathy [115, 118–121].
Elevated left heart filling pressures, a hallmark of (left-sided) heart failure [111, 122], are recognized to cause pulmonary venous hypertension (PvH) [123] irrespective of LV-EF [124]. This is attributed to a backward, downstream transmission of the elevated left-sided filling pressures, precipitating a rise in pulmonary venous pressure [6, 115, 118, 122]. An elevation of the pulmonary venous pressure directly elicits higher intrapulmonary vascular pressures, particularly of the PAP, and a decrease in pulmonary vascular compliance, hence stiffens the pulmonary artery(ies) [25, 98, 100]. Consecutively, RV afterload and RV systolic wall stress increase, potentially compromising RV function [25]. However, in early stages, PvH is not found to exhibit abnormal high pulmonary vascular resistance and thus does not cause pulmonary vasculopathy [96, 125].
As such, LHD: ↑ in LVEDP → ↑ LA-P → ↑ downstream pulmonary venous p → ↑ pulmonary p and ↓ pulmonary vascular compliance (respective PA stiffening) → ↑ PAP [14, 25, 98, 125].
Accordingly, although in most circumstances enhancements of the pulmonary artery pressure, PAP, are related to an increase in PVR, an increase in pulmonary pressures, namely mean PAP, and thus PH is not inevitably coupled with an increase in PVR [25, 125]. PH simply stands for elevated pressures in the pulmonary circulation rather than explicitly indicating pulmonary vascular alterations which are reflected by an elevated PVR [112, 118, 121]. However, PH may lead to increased PVR and to decreased pulmonary artery compliance [127]. A reduction in vascular compliance means an increase in vascular stiffness, which will cause a rise in vascular load on the upstream ventricle [96, 128–130]. RV-afterload is determined by the dynamic interplay between (1) pulmonary vascular resistance, (2) pulmonary vessel compliance, and (3) wave reflections [127], where PVR reflects the resistive RV-load component, and vascular compliance the pulsatile one [7]). Hence, PA stiffening also increases RV work load [127].
If PH is accompanied by a pathologic increase in PVR, adverse prognostic implications apply [131, 132].2
Anyhow, pathologically elevated pulmonary pressures, defined as a mean pulmonary arterial pressure ≥25 mmHg at rest, measured invasively by right heart catheterization [71, 126, 134], will impose an un-physiological pressure load (largely due to altered vascular properties) on the RV, provoking adaptive measures to face this burden potentially leading to right heart failure [14, 19, 20, 22, 23].
Physiologically, beat-to-beat variations in RV preload or afterload are addressed by adaption in right chamber dimension, applying Frank-Starling’s law of the heart: Abrupt, beat to-beat, increases in pre- or afterload are met with a mild rise in RV size, the so-called heterometric dimension adaptation (a diastolic effect), known as and described by Frank and Starling’s law of the heart [3, 135]. However, within a couple of minutes, starting already 20–30 s after the heterometric adaption applies, an increase in RV contractility, and as such a systolic adaptive effect, will firstly supplement but quickly completely replace the initial heterometric adjustment [3, 135]. This so-called homeometric reply, which does not require any pre-existing muscle or cellular hypertrophy [136], is referred to as “Anrep’s law of the heart [137]. It has been G von Anrep who demonstrated more than 100 years ago an intrinsically mediated increase in LV contractility in response to a raised LV afterload [137]. This reaction affecting the strength of contraction occurs independent of end-diastolic fibre length (the Frank–Starling–mechanism relies upon fibre stretch) or other extrinsic issues, like neuro-endocrine stimulation [138].
It has been challenged that the homeometric autoregulatory effect applies in vivo in the setting of a rapidly raised afterload since this mechanism has originally been demonstrated “only” in isolated muscle strips [139]. However, homeometric adaptation to afterload has been reported to apply in case the RV is exposed to pulmonary constriction if coronary perfusion is held constant [140]. Furthermore, some recent evidence even suggests that homeometric autoregulation may be the primary mechanism launched already for “initial” response and adaptation to brisk RV pressure load [141, 142].
However, physiologically, homeometric adaption replaces the heterometric adjustment after a few minutes as indicated by the chamber dimension, which returns to baseline after a few minutes, demonstrating the predominant role of homeometric (that is without chamber dilation), systolic function adaption in both acutely increased pre- and/or afterload [136].
Anyhow, up to 40% of RV systolic function, that means 2/3 of pressure and 80% of flow generation under healthy terms [143, 144], is derived from the LV systolic performance, largely from the septal oblique/helical orientated muscle fibre contraction, a feature referred to as systolic ventricular interdependence [14, 145, 146]. Of special note, these septal fibres, originating in the LV, reach up to the right ventricular outflow tract [147]. These bundles of muscle fibres functionally link RV and LV together and directly transmit contractile force generated by the LV to the RV [147, 148].
Oblique orientated myocardial muscle fibres are demonstrated to develop clearly more contractile power than transverse orientated ones [149], the latter typically found in the right ventricle [14]. RV dilatation, due to volume loading, increasing preload, or particularly due RV filling overload, as typically resulting from tricuspid regurgitation/insufficiency following RV dilatation, leads to a change in septal muscle fibre orientation, and the more transverse configuration implies loss of muscle strength [14]. This phenomenon is especially evident in LV systolic dysfunction where the naturally oblique orientated muscle fibres of the LV, and thus of the septum, take a gradually more and more transverse position, losing part of their power generation capacity, consecutively affecting RV systolic function as well [14]. On the other hand, an enhancement of the LV systolic function in the setting of a compromised RV function displays beneficial effects on RV performance [150].
Any rapid rise in pulmonary pressure (increase in PA input impedance) due to altered pulmonary vascular load, after-loading the right ventricle and/or a loss of RV contractility (altered myocardial properties, e.g. acute myocardial ischemia) causes an immediate increase in RV size, RV dilation, and concomitantly a rise in RV-end-diastolic filling volume (RVEDV) ensues [1, 3, 7, 16, 105–107]. However, RV adaption to PH is acknowledged to be decisively depend on the ventricle’s ability to adjust contractility in order to match the increased afterload the ventricle is facing in case of increased pulmonary vascular load [151, 152]. Accordingly, if the homeometric adaption is too short or even fails and cannot (fully) compensate for altered RV loading conditions, and/or if systolic RV (and/or LV) capabilities (contractile power) are suddenly lost (e.g. due to acute myocardial ischemia/infarction) [70, 74, 153, 154], heterometric measures persist or apply in addition, potentially able to meet (by applying the effects of the Frank-Starling-mechanism) the challenge imposed, although certainly at the cost of considerably increased RV dimensions (↑↑ RVEDV) [138, 155, 156]. This increase in RV size and filling is inevitably attended by increased right ventricular filling pressures (RVEDP) [23, 157–159].
If the RV dilates, it becomes a more cylindrical shape and thus the efficacy of the Frank-Starling-mechanism increases [141, 142]. However, under those circumstances, RV contractility is compromised (e.g. septal muscle fibre orientation, RV free wall performance) [14, 17, 133, 160], RV-EF impaired [31, 84, 161, 162], and RV pump failure and even cardiogenic shock may promptly ensue [89] if the compensatory mechanisms (most notably the increase in RV contractility as the predominant and physiological adaptive feature and alternative to match and face the elevated pressure load [135, 151, 152]) are insufficient and afterload mismatch persists [7]. As such, RV afterload has to be acknowledged as the major determinant of RV systolic function, and RV failure is commonly the result of increased RV afterload [14]. RV systolic function is much more than LV performance literally and crucially dependent on afterload [19, 163] (see Fig. 4.1).
Tricuspid regurgitation following RV enlargement may compound the conditions [1, 7], although they may also disclose a path to reduce RV afterload by offering a less restrictive way for the blood stream [164–166]. Furthermore, diastolic ventricular interaction (DVI) applies compromising left ventricular filling and thereby worsens global cardiac function and systemic circulation even more [83, 167, 168]. In any way, ventricular interactions (the ventricles are even more directly intertwined in malady [101]) play an important and critical role in RV-F pathobiology by taking a crucial impact on left heart and subsequently systemic cardiovascular function [145]. DVI, mediated by the pericardium and the interventricular septum (IVS) [1–3]), restricts left ventricular filling due to a leftward shift of the IVS in the presence of elevated pericardial constraint [1, 167, 169], thereby changing LV geometry [1, 170] resulting in impaired LV-contractility [3, 83]. Furthermore, due to the enhanced pericardial constraint resulting from RV-dilation, LV distensibility decreases, consecutively (as well) impeding LV filling, ultimately diminishing LV-SV [1, 3]. Subsequently, the compromised LV contractility may display considerable deleterious effects on RV systolic performance, systolic ventricular interaction, as about 1/3 (20–40%) of systolic RV pressure generation and up to 80% of RV flow generation [143, 144] results from LV contraction [145, 146, 171].
It is exactly ventriculo-arterial coupling which specifically refers to the relationship between ventricular contractility and afterload, in this case between the right ventricle and the pulmonary vascular tree [7]. As such, assessment of RV-PA coupling is a physiologic approach to this interrelated system [172]. Disrupted RV-PA-coupling is considered to contribute to progressive RV-malfunction [17]. RV-PA-uncoupling ensues as RV contractility does not match afterload [107, 173, 174]. Altered coupling may affect the efficiency of power transmission and thus dilutes blood flow output from RV to and within the pulmonary vessels, diminishing LV preload [22]. Indeed, recent studies report a reduced RV-PA coupling efficiency in different forms of PH [151, 175, 176]. In experimental tachycardiomyopathy RV-PA-uncoupling has been observed related to lack of a sufficient adaptive increase in RV contractility [177]. In a sepsis model with endotoxic-induced elevated PVR, initial preservation of RV-PA-coupling could not be maintained as the incipiently adaption in contractility did not persist [178]. On the other hand, several studies demonstrated preserved RV-PA-coupling in patients and animals with acute hypoxia related pulmonary vascular constriction (displaying acute RV pressure load), when RV contractility increased, matching the pulmonary artery input impedance [173, 174, 179, 180]. Accordingly, adaption of RV systolic function to increased pulmonary vascular load, causing PH, is necessary to maintain proper RV-PA-coupling. Uncoupling occurs in case of inflammation, long-lasting PH and left heart failure resulting in deficient RV contractile adaption (systolic ventricular interaction) [3].
The results consistently confirm the crucial role of homeometric adaption (incremental contractility) in case the RV is faced with a rapid or substantially raised afterload [151, 152]. RV-PA uncoupling is related to the onset of RV-failure and can be seen as an early sign of maladaption [181]. Deterioration of RV-PA coupling is associated with increased RVEDV [135], while the absence of increased RVEDV in the presence of raised pulmonary pressure indicates sufficient coupling [135].
As the hemodynamic aspects of the pathobiology of RV-F are decisively characterized by the alterations in RV pressures and volumes and the interventricular interactions it is worth and necessary to further discuss in depth volumes and pressures issues:
Both, brisk increases in RV afterload (e.g. a sudden rise in pulmonary pressures inducing PH, but substantial and/or prolonged enhancements in RV vascular loading conditions as well) and rapid and/or particularly considerable boosts in RV preload (e.g. quick volume loading) [7, 98] lead to a marked RV-dilation [1, 3, 7, 19, 23, 31, 106, 107] with increased right ventricular filling volumes (RVEDVs) [1, 3, 7, 19, 23, 31, 106, 107]. An increase in ventricular filling volume is in any case attended by a rise in filling pressure: “Acute increases in filling volumes yield higher filling pressures” [157]-a parallel upward shift of the PV-relation (see Chap. 1, Sect. 1.10). Acute volume loading of the RV or in case the RV dilates due to (abruptly) augmented RV afterload, both changes are enhancing RVEDV, and will thereby exert stress on the acutely literally indispensable pericardium which consecutively results in increased pericardial pressure and a noticeable parallel upward shift of the PV-relation, indicating that a higher absolute RV filling pressure is necessary to achieve a given RV filling volume [182]. As such, the increase in afterload itself exerts some impact on the position (parallel and upward) of the PV-relation. Hence, changes in vascular loading conditions result in parallel shifts of the diastolic PV-relation [157, 183] indicating alterations in pericardial and filling pressures (see chapter 1, section 1.10, extracardiac forces). Moreover, pericardial constraint will affect the thin-walled RV more than the LV, subsequently the increase in RVEDP is disproportionally higher than that in LVEDP [168, 184]. However, increasing pericardial constraint, as with increasing RV enlargement, results in less RV free wall stretch, limiting the effect of the Frank-Starling-mechanism [17].
Furthermore, the markedly enlarged RV size is accompanied by altered diastolic RV properties, shifting the RV diastolic pressure-volume-relation to a steeper proportion of the curve (leftward and upward shift) as changes in systolic load affect diastolic properties as well [17, 156, 185–189]. This denotes RV stiffening, and as such, PH stiffens the RV [190]. RV stiffness dilutes the RV free wall stretch, consecutively blunting the Frank-Starling-effect [17], increasing RVEDPs and central venous pressures [1, 83]. RV diastolic dysfunction and elevated RV filling pressures induce renal fluid retention (arginine vasopressin effect) [1]. Thus, several effects are contributing to the quite substantial increase in RVEDP when suddenly after loading the right ventricle.


The increase in RV-size has, due to the acutely literally nondistensible pericardium [191, 192], an impact on the LV [1, 2]:
- 1.
LVEDP will firstly increase due to the increase in pericardial constraint, as described.
- 2.
RV-enlargement leads, due to the restrictive properties (actually literally not distensible) of the pericardium and the limited space within the pericardial sack, to a competition of the two chambers for space resulting in a reduction in LV-preload [135]. This reduces LV-filling, a series effect as the two chambers are arranged in a row [169, 193, 194].
- 3.
The compromised RV systolic properties ejecting a lesser extent of blood into the pulmonary circulation [14, 17, 133, 160] and RV-PA-uncoupling losing further energy, resulting in a loss of flow output [107, 173, 174] are both contributing to the shorted LV preload. Both issues may be interpret within the scope of and referred to as series effects.
- 4.
Due to largely diastolic ventricular interaction, mediated by the shared structures of the ventricles (pericardium and the interventricular septum (IVS) [159, 191, 192]), the IVS will be shifted in the presence of increased pericardial constraint towards the LV cavity thereby changing LV geometry [1], compressing the left chamber subsequently impairing LV filling, and leading to impaired LV contraction [1–3]—and consecutively also to diluted RV contractile power. DVI, coming in general and particularly into effect with increasing RVEDP [72, 169], essentially contributes to acute right heart failure pathobiology and makes a crucial hemodynamic impact on right and left heart and subsequently systemic cardiovascular function [1, 145, 169].
- 5.
LV diastolic properties are altered, largely an effect of DVI [195] and due to the increasing RV size and RVEDP, causing LV diastolic dysfunction: The LV becomes stiffer (reduced LV compliance) [185, 195–199], resulting in an increase in LVEDP (leftward and upward shift of the LV PV-relation) and may cause a reduction of LV-filling and consecutively diluted LV-SV [1, 3, 185, 197, 198].
Hence in summary, LV size will substantially decline (LVEDV ↓↓) while LVEDP will increase (LVEDP ↑↑)3 and LV systolic capabilities will be diminished, as depicted by the following causal chain.


Noteworthy, RV accommodates much better and quicker to changes in preload (e.g. volume load) compared to the very poor tolerance of sudden (and/or substantial and/or prolonged) increases in afterload (pressure load) [19, 20, 100, 200]. In contrast to pressure overload, the right ventricle tolerates primary volume overload conditions over a long period quite well as evidenced by the clinical courses of patients suffering from intracardiac shunts (e.g. Eisenmenger’s syndrome), and tricuspid or pulmonary regurgitation [15, 17]. This may be due to:
- (I)
RV volume overload does not relevantly impair contractile dysfunction [57],
- (II)
The RV is preconditioned to tolerate volume loading in the foetal period, and in case of congenital abnormalities, foetal right ventricular phenotype properties may persist [201, 202]. Furthermore, patients with Eisenmenger’s syndrome decompensate if pulmonary vasculopathy and thus an afterload burden develop or shunt reverses [15].
Insofar, even acute volume loading alone will not induce predominantly acute right heart failure in otherwise reasonably normal hemodynamic conditions. However, acute and rapid or extensive volume loading, in particular over a certain limit [198] is reported to potentially cause transient RV-dilation in special circumstances [199, 203]. Volume loading should always be referred to as “pre-loading” the ventricle: In this respect, pre-load may be defined as the combination of all factors contributing to passive end-diastolic ventricular wall stress [7]. RV preload is determined by volume and pressure prior to contraction. Respiratory alterations affect the RV filling and the pericardium constrains the thinner, low-pressure RV more than the high-pressure LV [98].
Aside the more specific hemodynamic factors and features, further issues are demonstrated to significantly influence and contribute to the pathobiology of acute right heart failure:
A markedly enhanced activation of the neuro-endocrine and the immunologic/inflammatory-endothelial cascades displays a variety of functional alterations, particularly endothelial dysfunction (ED): Adrenaline and noradrenaline, angiotensin II (the most bioactive representative of the renin-angiotensin-aldosteron-system), cytokines, endothelin-1 in the presence of an altered NO metabolism and availability (a constellation typically indicative for ED), and natriuretic peptides (with their potential to counterbalance to some degree the effects of the aforementioned agents), are released and secreted, offering compensatory input, and as such are involved in and contributing to the pathophysiology of acute RV-D/RV-F [1, 83, 91, 204–211]. The release and discharge of adrenergic substances with positive inotropic and chronotropic effects may facilitate the contractile efforts [106], however, net contractility may be acutely even reduced [107].
These compensatory mechanisms applied with their predominantly pulmonary and systemic vasoconstrictive properties improve pulmonary blood flow and may temporarily stabilize the pulmonary and systemic hemodynamics [212], but are gradually maladaptive [27, 213, 214]. However, the increase in RV size and pressures lead to increased wall tension and cardiomyocyte stretch [106], consecutively the coronary perfusion is affected and a higher oxygen demand and consumption is displayed, potentially leading to RV ischemia [107, 215], at least if no effective reduction of RV afterload can be achieved [216]. In case of acute pulmonary embolism, causally responsible for the abrupt rise in afterload, RV ischemia is demonstrated to be of pathophysiological significance in the acute phase [217, 218]. Elevated RVEDPs and considerably diminished blood pressure not matching the metabolic demands may cause RV ischemia and compromised RV contractility [219]. Nevertheless, study results are inconsistent in regard to what degree ischemia is responsible for and contributes to RV contractile malfunction [220–222]. Moreover, myocardial stunning (even in case of RV-AMI) rather than true cardiomyocyte loss is suggested to underlie progressive contractile impairment [195].
Furthermore, there is some evidence suggesting that “just” pressure overload itself may down-regulate RV contractility [22, 223, 224]. In the absence of ischemia, activation of intracellular paths affecting the contraction sequence and procedure [225, 226], activation of apoptosis [227, 228] or even disturbed NO-pathways due to endothelial dysfunction may be involved.
As RV contraction will be prolonged (since myocytes prolong under stress contraction time action potential duration [7]) in case of RV strain, blood is still ejected into the pulmonary vessel system while the left ventricle already resides in diastole, the interventricular septum shifts to the left side in the late systole [229, 230] restricting and reducing LV-space [106, 107, 216]. This desynchronization of both ventricles will aggravate RV malfunction [7]. Dys-synchrony is reported to arise early on during the adaptive process, intended to support systolic function of the RV, however, this implies that LV-filling is blunted already early in the course [2].
If the hemodynamic compromise cannot be stabilized, as both, the (supplementary) heterometric efforts and especially the RV contractile power (homeometric adaption) are together not able to generate the performance necessary to match the acute increase in PA-input impedance (and/or the exposure of the RV to acute afterload mismatch persists), acute RV failure applies and may rapidly end up catastrophic with a circulatory collapse [95, 96, 135]. The progressive RV-dilatation and the accompanying, considerably elevated (and further rising) right ventricular filling pressure, reflecting and indicating RV-dysfunction/failure [25], may induce a vicious cycle ending up in circulatory collapse [135]. Even a mild acute elevation in pulmonary pressure eliciting mild PH may cause a substantial drop in RV-SV [231, 232]. Blunted RV-SV and thus reduced LV preload delivery (due to diminished SV generated by the weak RV [169, 194, 233] and due to RV-PA-uncoupling [107], both effects may be referred as to series-effects [169, 194, 233]), the excessive LV compression (largely due to DVI [135]), and the LV diastolic dysfunction (and therefore impeded LV distensibility) [81, 234], result in a marked LV underfilling [2, 3, 83] and a considerably impaired LV systolic function [2, 3, 83, 197, 198, 235].The combination of LV underfilling and compromised LV systolic function may inevitably precipitate hypotension and systemic hypoperfusion (adapted from Zochios, [93]). This will result in even less contractile support for the RV, while hypotension potentially dilutes right and left coronary perfusion contributing to circulatory collapse [107, 216, 236]. However, as discussed above, other, non-ischemic issues may contribute to the now progressively deteriorating RV systolic properties [22, 223, 224, 226, 228]. Though, “RV failure begets RV failure” leading into a progressive downward spiral of worsening myocardial dysfunction and incipient shock [93].
Once systemic pressure, e.g. MAP, begins to fall, hemodynamic collapse will ensue rapidly. As depicted in Fig. 4.2, a work by Guyton [89], the hemodynamic range within the disastrous malady course develops may be very narrow. Patients, of course with symptoms and signs of RV-F, although appearing to be in a clinically reasonable and stable condition with acceptable BP, but with no obvious evidence of relevant hypoperfusion, and only mild to moderately elevated CVP, may decompensate immediately and unexpected: Compensatory mechanisms may be already exhausted, but this is not recognized as clinical and hemodynamic features are still tolerable. Furthermore, no additional features (such as ischemia), typically aggravating the malady, may be observable. Nevertheless, issues including non-ischemic related paths [225, 227, 228], stunning myocardium [195] or RV-pressure overload associated, the contractile forces down-regulating mechanisms [22, 223, 237], and, hopefully not, therapeutic measures such as very cautious volume application (assuming a still available preload reserve in order to ameliorate rather than to destabilize the situation) may be the trigger of the disaster by initiating MAP to fall. Insofar, circulatory collapse may insert abrupt and quite unexpected in otherwise hemodynamically stable appearing patients.



Fig. 4.2
At some point (A), RAP/CVP starts to increase with increasing pulmonary pressure/increasing RV-afterload. The increase in filling pressure allows for recruitment of contractile reserve via Frank-Starling-mechanism (heterometric adaption). However, this compensatory action of this crucially afterload-dependent right ventricle will soon reach a limit (point B), where systemic pressure is going to abruptly drop and circulatory collapse applies. As there is only a very small range between onset of CVP/ RVEDP increase and collapse (see difference between point A and B), caution is advised (e.g. to apply fluids), once CVP is elevated. There is no indicator notifying imminent circulatory catastrophe. Modified from Guyton AC. Circ Res 1954;2: 326–332 [89]. With permission

Fig. 4.3
Overview of the pathophysiology of right heart decompensation and failure: the diagram summarizes the most relevant pathobiological and pathophysiological features and sequences of acute right heart failure. It is based on publications by Price [16], Schwartz [14], Naeije [3], Vonk-Noordergraaf [7], Teichman SCCM 34th congress 15–19th Jan 2005, and Kucher Acute Cardiac Care Meeting of the Esc Prague 23rd Oct 2006. TI tricuspid insufficiency, BP blood pressure, DVI diastolic ventricular interaction, PH pulmonary hypertension
In case of a gradual increase in PAP and/or PVR as usual in LHD, the so-called homeometric contractility adapation to afterload according to Anrep’s law [137] may ensue [135]. The homeometric adaption and remodelling is characterized by an increase in ventricular systolic function (e.g. contractility) without chamber dilatation in order to meet the load the ventricle is facing [138]: The right ventricle adapts to the increased afterload by increasing its wall thickness and contractility [7]. Homeometric adaption is shown to be the predominant feature of RV to face and to adapt to increased afterload and to ensure preserved RV-PA-coupling [3, 135].
Indeed, in case of gradual increases of pulmonary pressures or due to mild/moderately but chronically increased pulmonary pressures, RV develops a hypertrophy and thus concomitantly adapts [96, 151]. The initially enlarged RV end-diastolic volume triggers the development of RV hypertrophy enhancing contractile capabilities and thus adapts to the new challenge, maintaining RV-SV by increased contractile force [216]. In animal models, hypertrophy is recognized already 96 h after the onset of increased afterload [238]. This is principally confirmed by studies in humans suffering from ARDS where already after 2 days of PH (ARDS and mechanical ventilation cause an increase in transpulmonary pressure which correlates with the magnitude of RV afterload [95]), a moderate thickness of the free RV wall could be demonstrated [31]. Hypertrophy will reduce wall tension (LaPlace), and the interventricular septum, initially bulging to the left (D-shaping), flattens [216]. Notably, although the RV elastance may rise two-to three-fold during the acute phase, no acute systolic RV dysfunction has been reported [141, 142]—accordingly the enhanced RV–elastance indicates true augmentation in contractility. Moreover, some degree of RV-dilation establishing heterometric, dimensional adaptation via Frank-Starling-mechanism will be implemented as well [2].
However, if the load rises further, becoming too high for a too long period, or if these compensatory mechanisms are insufficient to match the load imposed, RV-PA uncoupling associated with (further) increased RVEDV occurs [135, 138], a heterometric adaptive mechanism, indicating RV dysfunction [7], or even RV-failure [3, 135]. Severe inflammatory conditions (e.g. septicaemia), long-term increase in PVR or advanced heart failure are disorders predisposed for RV-PA uncoupling and RV-dysfunction [3, 135]. Furthermore, even the described remodelling may, after many years of compensation, progress to chamber dilatation, consecutive tricuspid insufficiency, and frank RV-failure [172]. It may be speculated that pressure overload downregulates RV contractility [22, 223, 224] and thus later in the course, heterometric compensation will become necessary due to decelerating contractility. This is in line with recent study results, showing that patients with long-standing volume overload conditions, although compensated and most often only marginally symptomatic over many years, nevertheless carry an increased risk for cardiac morbidity and mortality [239, 240]. Other precipitating factors discussed include ischemia, as RV hypertrophy potentially decreases RV subendocardial perfusion, while the arising RV-dilatation entails increased wall stress and thus a higher oxygen demand [96] and neurohormonal/inflammatory issues [241–245].
Acute and chronic RV failure, being attended by enhanced neurohormonal discharge and sodium and water retention, is thereby consecutively accompanied by elevated CVPs [1, 24] which may exert deleterious effects: Increased CVP impairs lung lymphatic drainage, leading to interstitial pulmonary fluid accumulation causing shortened lung compliance, impaired gas exchange, and promotes the development of pleural effusion [5, 246]. Renal venous pressure is subsequently increased and provokes cardiorenal syndrome [5, 247, 248]. Hepatic and intestinal congestion occurs facilitating cholestasis and ascites development [1, 5], impairs gut absorption and may allow for translocation of gut microbes into the blood stream [96].
4.3.1.1 To Sum Up (see Fig. 4.3)
Acute right heart failure is a complex, heterogeneous clinical syndrome of miscellaneous aetiologies [1, 2, 93, 96]. LHD is by far the most common reason causing acute RV-F [27, 28]. Any acute (rapid) increase in pulmonary vascular pressures imposing a (additional) load on the RV, precipitating an afterload mismatch, may provoke acute RV-F [14, 27–29, 95, 96, 135], and even mild increases in PAP are reported to potentially trigger acute right heart failure [231, 232].
Acute RV failure is characterized by RV dilatation [1, 3, 7, 19, 105–107], generally attended by increased right ventricular filling pressures [157–159, 186], and impaired RV contractile properties [2, 3, 14, 17, 160, 197] in the presence of clinical signs, foremost dyspnoea and fatigue, as well usually fluid accumulation and edema formation, of RV dysfunction, furthermore evidenced by elevated CVP (RA-P/RVEDP) [1–3, 5, 7, 24, 25, 246, 247], often accompanied by organ, particularly renal, dysfunction [5, 24, 123, 246–248].
Acute RV-F arises if the load imposed on the RV, generally after-loading (but pre-loading principally may affect the RV as well) the right ventricle, cannot be met and counterbalanced by an appropriate increase in RV contractile power [95, 96, 135], referred to as homeometric adaption [137, 138]. Deficient RV systolic performance may be subject to impaired RV and/or LV contractile capabilities [3, 7, 16, 83, 105, 106, 143, 144], but is usually attributed to the brisk (and/or substantial) rise in afterload [1, 7, 14, 15] hitting a ventricle which is anatomically and functionally not designed and not evolved to deal with high pressure loads [19, 105–107, 200] and whose performance is literally crucially dependent on afterload [19, 163]. Adaption to pressure load can only succeed if the ventricle is able to strengthen its contractile capabilities [151, 152].
Neuro-endocrine and inflammatory-endothelial measures and replies support and govern in a sense the compensatory activities [1, 83, 91, 204, 206–211], but may turn to be maladaptive over time (e.g. fluid retention) [213, 214].
Anyway, in the case the homeometric adaptive efforts are too little or fail, substantial RV enlargement, accompanied by elevated RVEDP, immediately ensues [1, 3, 7, 16, 19, 105–107, 200]. This enlargement is an attempt to improve RV performance by applying the Frank-Starling-mechanism, facilitating blood ejection, output and blood flow [172, 173]. However, this approach has transpired to induce a series of potentially deleterious, although basically compensatory, measures and reactions, which are largely related to ventricular interactions [14, 72, 107, 145, 167–169, 173, 174]. These arrangements will substantially affect the LV resulting in diminished LV size, and LV diastolic and LV systolic malfunction [2, 3, 81, 83, 156, 196–199, 234]. Subsequently, hypotension, a jeopardized systemic circulation with lurking organ and tissue hypoperfusion and an even more compromised RV function are to follow, potentially ending up in circulatory collapse and shock [93, 95, 96, 135].
Of note, some correlations, relationships, interrelations and causative interconnections |
(a) PVR is calculated by the ratio of the transpulmonary pressure to the transpulmonary flow [249]: |
PVR = PAPmean/SV × HR(SV × HR = CO) |
↓ |
• ↑ Heart Rate (often the first attempt to compensate acute RV pressure and/or volume load [250]) |
4.3.2 Special Pathophysiological Issues
4.3.2.1 Diastolic Ventricular Interaction
The global hemodynamic consequences of RV-D are dependent on the critical interaction between the two ventricles [251, 252]. Under physiological conditions we will find similar end-diastolic volumes in RV and LV [31, 253]. The heart chambers are enclosed by the pericardium and share the interventricular septum and, as such, ventricular interactions occur [171, 254, 255].
“Diastolic ventricular interaction (DVI) refers to competition for space within the non-distensible pericardial sack when RV dilates” [135]. Changes (particularly sudden changes [168, 171, 254, 256]) in the end-diastolic volume (and intraventricular pressure) of one ventricle will directly influence the volume and intraventricular pressure and thus compliance [256] of the other ventricle [168, 169, 254].
These diastolic interactions are mediated via the shared structures of the ventricles, the interventricular septum and the pericardium with its constraining effects on ventricular filling through poor distensibility [159, 191, 192]. Thus, an increase in the cross-sectional area of one ventricle, i.e. due to volume loading or enlargement, necessarily reduces the area of the opposite ventricle (resulting in less filling volume), and may simultaneously affect the pericardial pressure (PP) [72, 159]. The total cardiac volume (filling) remains unchanged [159, 257]. Therefore the pericardium plays a key role in the loading conditions [157, 258] and this is particularly seen in the acute situation.
The increase in RVEDV, which is accompanied by a rise in RVEDP and PP, shifts the interventricular septum towards the LV cavity. This occurs subject to the restrictions imposed by the acutely non distensible pericardium on the RV as the RV-cavity size increases [9, 196].
Furthermore, Kingma showed that in acute RV pressure or volume load (increased RV preload [7, 98]) the interventricular septum becomes flattened or even concave at end-diastole due to RV dilatation and raised RVEDP, diminishing the trans-septal pressure gradient (trans-septal pressure gradient = LVEDP − RVEDP [259]) and pushing the septum towards the left ventricle [259]. Numerous publications confirm the change in the septum position in different conditions such as acute and chronic pulmonary hypertension [168, 184], congestive heart failure [72, 254] and mechanical ventilation [260]. The leftward shift of the septum and the constraining effects of the pericardium compress the LV with a resultant decrease in LV-size and in end-diastolic LV-filling (reduced LVEDV) [9, 196, 261], producing a reduction in LV-SV [262, 263]. Furthermore, the LV diastolic properties are affected as well, and the reduction in LV compliance in so far contributes to the compromised LV-filling and, hence, the reduction in LV-SV [9, 196, 199, 264]: This is due to the flattening of the septum as RV dilates and as the RVEDP rises, subsequently affecting LV compliance [9, 197, 199], and thus resulting in altered LV diastolic function, diastolic dysfunction, with abnormal LV relaxation and reduced LV compliance [9, 196, 197, 199].

4.3.2.2 The Role of the Pericardium in Diastolic-Ventricular Interaction
The constraining effect of the pericardium not only limits the LV-filling but also the dilatation and filling of the RV: Under normal conditions RVEDP and PP are low, with the natural pericardium contributing by 30–40% to the total RV end-diastolic filling pressure [268]. But in cases of raised intra-thoracic pressures [10, 42–44, 103, 153, 154] and/or (otherwise) altered pulmonary hemodynamics [9, 30, 156, 159, 198, 257, 269, 270], features typically associated with changes in RV loading conditions [21, 120, 251, 252, 269, 271, 272], “external” pressure is exerted on the heart [46, 273–275], exhibiting a noticeable constraining effect by the pericardium particularly on the thin walled RV [168, 184]. Both RVEDP and LVEDP will rise, but the rise affects the RVEDP more than the LVEDP (↑ RVEDP > ↑ LVEDP) [46, 168, 184]. In regard to DVI, changes in filling pressure are more pronounced in the RV than in the LV and thus volume loading would increase RVEDP more than LVEDP, whilst for unloading the fall in RVEDP exceeds the fall in LVEDP [72, 168, 254, 271]. Right-sided HF always implies an increased PP [276] and thus constraint should always be considered in case elevated PPs are commonly present.
Ventricular interaction due to pericardial constraint is diminished as long as the PP is <5 mm Hg [277]. In the thin walled RV, if RVEDP ≥ 4 mm Hg, PP will increase in a parallel fashion [278]. A PP exceeding 9–10 mm Hg will exert substantial constraint on ventricular filling [273, 278]. When LVEDP exceeds 10–15 mm Hg, the LVEDP-LVEDV relation becomes much steeper and the pericardium limits further increases in LV end-diastolic volume [279, 280].
As discussed in Chap. 1, the CVP reflects the pericardial pressure [281, 282], and pericardial constraint accounts for 96% of the RA pressure, if CVP > 10 mm Hg [273]. The ability to maintain an adequate RV-SV by RV-dilatation is very limited. RV-SV decreases almost linearly with an abrupt increase in afterload as soon as pulmonary hypertension (mean PAP ≥ 25 mm Hg) occurs, despite all compensatory attempts (RV-dilatation) [283]. Very soon the constraint exerted by the pericardium will restrict the dilatation and further fluid administration in order to increase RVEDV and thus ensure a proper RV-SV is, if at all, only of marginal help. Contrary to previous belief, fluid administration will be harmful because any further dilation of the RV cannot correct the LV-filling deficit and may reduce LV-filling even more [66, 168, 169, 184, 284–286]. If RV-D occurs, no further fluid administration is advisable, volume loading will be harmful [287, 288] in the failing RV: In case of increased RV filling pressures above 10–15 mmHg, fluid loading should be avoided because volume application may worsen the hemodynamic situation by enhanced pericardial constraint including a further shift of the interventricular septum towards the left ventricle [7]. Conversely, volume unloading will be beneficial and allows for an increase in SV/CO [287, 288].
4.3.2.3 Auto-aggravation
RV-dilatation (RVEDV ↑) and the alteration of the RV-geometry secondary to the increased RV-afterload or substantial volume loading leads to a tricuspid annulus dilatation and functional tricuspid insufficiency (TR) [289–291] which is further aggravated by the increased RVEDP [9, 289]. The tricuspid regurgitation leads to congestion in the hepatic and renal vascular bed and to a fall in RV-SV [9, 289] which is, as per definition, RV-F. Less blood volume will be ejected into the pulmonary vasculature due to the fact that the PA-pressure is higher than that on the venous side and, due to the TR, ejection into the low pressure conduit is easier. The reduced RV-SV implicates a further (additional to the reduction of LV filling secondary to the DVI effect) reduction in LV preload via the so called series effect [194, 292].
4.3.2.4 Series Effect
The two ventricles are coupled in a row (series), one after the other, and thus their output necessarily is equal over time [169, 193]. Therefore, a reduction in right ventricular output results in less blood (volume) being transported to the LV [194, 292]. Less filling of the LV (less LV-pre-load) will result in a fall in LV-SV as per the Frank-Starling mechanism [262, 263]. ‘The performance of the RV determines LV-preload’ [193].
Due to systemic vasoconstriction the systemic arterial BP is usually maintained in the initial phase of acute RHF [293]; however, with a further, substantial decrease in LV preload causing considerable loss of LV-SV, a BP drop is inevitable [289, 294, 295]. RV-F is often accompanied by hypotension [251, 296]. Kerbaul [22, 160] and Bellamy [297] showed that, unfortunately in this situation, we cannot expect an increase in contractility to maintain or increase the RV-SV.
The combination of autoaggravation and the series effect can be summarized below:
4.3.2.5 Pulmonary Hypertension and Ischemia
An elevated PA-pressure puts the RV at risk of myocardial ischemia [33, 133, 298], with or without pre-existing coronary artery disease [299, 300] and RV-F may occur as a result of the ischemia [301]. RV-dilatation increases the likelihood that ischemia will develop because, at a certain point, a critical increase in wall tension and stress (secondary to RV enlargement) occurs, producing a significant mismatch between oxygen supply and demand [289].
With an increase in RV-afterload, the isovolumetric contraction phase and ejection time are prolonged and an increase in RV myocardial oxygen consumption results [9, 161, 221]. An increased oxygen demand would normally be compensated by a substantial increase in RCA-perfusion [221], but, in cases of low RCA perfusion, there is a risk that incipient RV myocardial ischaemia will further worsen the RV-function [221, 251, 284, 294]. As RV-F is often accompanied by hypotension, predominantly secondary to the reduction in LV-SV as described above, resulting in a marked reduction in myocardial perfusion [221, 296, 301, 302] a worst case scenario may occur, the combination of PH and ischaemia [221, 284, 294, 303].
However, recent study results fundamentally challenge the described role of ischemia in the context of PH and (acute) right heart failure, rather conceding ischemic cell destruction being the last and decisive step in the deleterious disease course only in special cases such as acute pulmonary embolism [304, 305]: The results are suggestive of myocardial stunning rather than true cardiomyocyte loss causing progressive contractile impairment [306]. Moreover, progressive contractile dysfunction may be even caused by non-ischemic issues as some authors suspect [81, 307, 308]. Moreover, it is not at least the RV pressure overload itself which is suggested to down-regulate and thus to contribute to the progressive deterioration of RV contractility [81, 307, 309].
Hence, the development of an ischemic right ventricular myocardium [30, 33, 284, 294, 301, 302] may be in some conditions, e.g. pulmonary embolism [304], the final step in the pathophysiological cascade of RV-F where life threatening heart failure will almost inevitably develop [30, 284, 294, 303].
Ischemia of the right ventricular myocardium occurs when RCA-perfusion pressure <25–30 mm Hg [301, 302]; in the case of PH, the RCA-perfusion pressure has to be >45 mm Hg in order to avoid ischemia [302] and, if a significant RCA stenosis is present, an even higher perfusion pressure is required [30, 236, 301].
4.3.2.6 The Interventricular Septum and the Apex
In critical situations such as acute RV pressure or volume load, and particularly when RV ischemia develops, the interventricular septum (IVS) ‘behaves’ as a functional part of the RV [310, 311]. In case of acute RV pressure or volume load, the IVS moves during systole towards the RV in a ‘paradoxical’ fashion. This ‘paradoxical’ septal movement is an active process of the interventricular septum at the end of systole allowing prolongation of the RV contraction phase, whilst the LV starts to relax [31], moving towards the RV-cavity and increasing the RV contractile force [31, 312]. The loss of the contractility of the septum under such conditions will markedly worsen the haemodynamic situation [251], but inotropic drugs in this situation may augment the RV systolic function by improving the contractility of the IVS [311, 313, 314].
Furthermore, the contraction of the apex of the heart contributes in cases of RV-D/RV-F to the net contractility of the right ventricle as well [311, 315].
Therefore if either the septum or apex fails, e.g., myocardial infarction, the decrease in LV contractility may result in RV-F [316].
The functional behaviour described above is in accordance with the anatomy. The shared pericardium and septum, the mutually encircling epicardial fibres, and the attachment of the RV free wall to the anterior and posterior parts of the septum allow the apex and the septum to make a contribution to systolic RV function [15].
4.3.2.7 The Left Ventricle
As described, the left and right ventricles are inter-related. LV dysfunction/failure affects RV-function, leading to RV-D/RV-F in several ways. LV-dysfunction may increase the RV-afterload due to pulmonary congestion [15], and/or because of a reduced MAP, the RCA perfusion may decrease, leading to RV-ischaemia [317]. However, LV-dysfunction also exerts an influence on lung mechanics and gas exchange [318], with a reduction in lung volume and lung compliance [319, 320], consecutively potentially affecting RV pre- and/or afterload [321].
Conversely, RV pressure overload may affect LV properties such that pulmonary congestion/edema, indicating LV dysfunction, may arise in a primary normal LV [32].
4.3.2.8 Mechanical Ventilation
Mechanical (positive pressure) ventilation [33, 42–44, 322, 323] and the application of PEEP [35–38, 47–50, 52, 324, 325] increase the intrathoracic pressure (pleural pressure). Artucio [326] and Brienza [327] demonstrated that the application of PEEP and/or positive pressure ventilation may lead to a rise in transpulmonary pressure and an increase in RV-outflow impedance [42–44]. Increasing tidal volumes raises intrathoracic pressure [42, 45] resulting in a marked elevation of the transpulmonary pressure with the potential risk to cause an acute cor pulmonale as found in a substantial number of patients [328]. Transpulmonary pressure directly correlates with RV-afterload [45] and since transpulmonary pressure rises in positive pressure ventilation and PEEP use, RV-outflow impedance will increase [46, 320, 329], which may promote the development of RV-D.
RV-function may also be compromised via another mechanism:
With increasing pleural (intrathoracic) pressure, we find an impairment of LV- and RV-compliance: RV-compliance decreases markedly with only small increases in pleural pressure whilst the LV-compliance decreases a significant amount only with higher increases in pleural pressure [46, 51]. As a consequence, the steep rise in RVEDP associated with only very small increases in RV end-diastolic filling [46] is accompanied by a parallel rise in PP with the potential to cause DVI (see Sect. 1.8 of Chap. 1 and DVI of this Chapter).
Pleural pressure is directly transmitted to the pericardial space [330] and so an increase in pleural pressure will increase the PP. Therefore, the normally low RVEDP and PP will rise markedly in mechanical ventilation, pneumonia, ARDS, etc. and so will contribute to an ↑ in the pressure surrounding the heart [331]. Any rise in pleural pressure will, via a concomitant rise in PP, limit the distending capacity of the cardiac cavities and will exert a constraining effect on both RV and, to a lesser extent, on the LV [51].
Furthermore, with mechanical ventilation the venous return is compromised, reducing the RV-filling and function and will hence reduce the RV-SV [332].
However, positive pressure ventilation and PEEP are not always detrimental. There is evidence that relatively low PEEP levels (≤8–10 cm H2O) have beneficial effects on the pulmonary haemodynamics and do not increase the RV-afterload significantly, even though the pleural pressure and thus the transpulmonary pressure are elevated [52]. Schmitt [333] found that the use of a low PEEP improved the blood flow through the pulmonary vessel bed, reducing the RV-afterload and the risk of RV-D. The reasons behind these beneficial effects are:
Air (gas) trapping is often present in respiratory failure due to chest infection or ARDS and increases the pleural pressure, the trans-pulmonary pressure, and the pulmonary vascular resistance. Gas trapping is relieved by (low) PEEP, hence reducing transpulmonary pressure and improving blood flow through a reduction in pulmonary vascular resistance [51, 334];
(Low) PEEP is beneficial in diseased and stiff lungs/lung compartments as it improves blood flow in the pulmonary vascular bed [51, 333–335]. Interestingly the PEEP-levels mentioned above, which are beneficial for pulmonary haemodynamics, correspond to those called ‘best PEEP’ described by Sutter in 1975 [335]. He found PEEP levels around 8 ± 4 cm H2O resulted in optimal oxygenation transport in ARDS patients. So, these PEEP levels seem to be beneficial for both the treatment of the respiratory failure and the maintenance of a sufficient cardiac function. There is no doubt, however, that PEEP levels >10–12 cm H2O exert a significant RV pressure load (increased RV-afterload) and cause a leftward shift of the interventricular septum [51];

However, it has to be stressed that, in case of pre-existing and/or manifest RV-D/RV-F, PEEP was found to increase RV-afterload in every case and may worsen the hemodynamic situation by its net effect [40].
Meanwhile, a balanced lung- and “heart” protective approach has been proposed, essentially limiting the plateau pressure within the airways to <27 cm H2O, best complying with the necessary requirements [81, 340–343]. If needed, mechanical ventilation with low tidal volumes (6(−8) mL/kg predicted body weight [344–347]) and relatively low PEEP (8–12 cm H2O) is appropriate in patients with pulmonary hypertension [21, 348].
4.4 Diagnostic Aspects
4.4.1 Clinical Features
Cardinal clinical manifestations of RHF are exercise limitation and fluid retention [7]. Exercise limitation is the earliest sign of RHF and is a strong predictor of survival [349–351]. Exercise limitation is related to a decrease in flow reserve during physical stress [352–354]. Further, a reduction in peripheral blood flow can increase lactate production, contributing to muscle fatigue. Supraventricular tachycardia may contribute as well [355]. Syncope is a less common symptom often indicating severe limitation in flow reserve. RV failure may further lead to chronic kidney disease and hyopnatremia [356]. Congestive hepathopathy is often observed in patients with RHF and PAH, cirrhosis is a late complication.
Hemodynamically, acute RV decompensation is characterized by enlarged RV size with enhanced end-diastolic filling volume attended by an increase in RVEDP (acute increases in filling volumes yielded higher filling pressures [157]), RV diastolic dysfunction [156, 185], and diminished and falling CO [185]. Some patients with severe and progressive RV-F may even expire normal pulmonary pressures due to marked reduction in CO [1].Thus, the interpretation of PAP has to consider CO and severity of heart failure.
Patients presenting with acute decompensations of chronic PH can often clinically be barely distinguished from those with acute RV-F attributed to acute PE, as clinical presentations are very similar [1].
As such, although there are a lack of specific clinical signs in acute right heart dysfunction or failure [357] but, nevertheless, the following features are suggestive of acute RHF and may be present [9]:
• Neck vein distension | • Hepato-/hepato-splenomegaly |
• Positive hepato-jugular reflex | • Abdominal discomfort |
• Renal impairment with oligo-anuria | • Hypotension |
• Tachypnoea is present in up to 80% [358] | • Peripheral oedemaa |
• Evated lactate, disturbed coagulation and raised liver enzymes may by an expression of liver dysfunction due to hepatic congestion [357] |
The clinical presentation is furthermore markedly influenced and determined by the underlying source precipitating RV-F and existing comorbidities [83, 144].
To conclude, acknowledged clinical cardinal signs of RV-F include [1]
- (a)
Fluid retention potentially causing peripheral edema, ascites and anasarca,
- (b)
Limited systolic reserve or low cardiac output leading to and provoking exercise intolerance and fatigue,
- (c)
Atrial and ventricular arrhythmias.
4.4.2 Serum Biomarkers
BNP has a strong, positive correlation to PVR and RVEDP in patients suffering from primary pulmonary hypertension [360, 361]. BNP rises gradually with increasing severity of RV-D/RV-F [306, 362, 363]. However, the thresholds of when to diagnose RV-D (RV-F) are still in discussion and vary from between >50 pg/mL [364] and >100 pg/mL [365]. Furthermore, elevated BNP levels may be present in chronic RV-D and chronic PH [208, 361, 366].
Troponin I > 0.1 μg/L (pathologically elevated) was found only in severe RV-D caused by pulmonary embolism [364]. Its occurrence is associated with early mortality [367, 368]. In the case of pulmonary embolism, patients with a negative serum troponin and normal ECG are at the lowest risk [369].
Both, Troponin and BNP have excellent negative predictive value and tend to exclude a complicated hospital stay when negative on admission [370, 371].
However, as both cardiac markers are not specific for right ventricular issues at all, the interpretation of their results can only be done in the clinical context they occur [2].
4.4.3 Electrocardiography
ECG ST-elevation (>0.1 mV) in VR3 and/or VR4 in patients with inferior ST-elevation acute myocardial infarction is highly specific for RV-ischaemia due to a proximal RCA-lesion (sensitivity 83%, specificity 77%) [64, 74]. Involvement of the RV, as a complication of acute inferior myocardial infarction (ST-elevation in II, III, aVF [372]) is to be expected in approximately 50% [70].
4.4.4 Echocardiography
Direct pressure and volume measurements can be made using a Swan-Ganz-conductance catheter [373]. Although right heart catheterisation has previously been the method of choice, echocardiography, due to favourable comparisons to the catheter results and as the less invasive method, is now widely used [374]. An echocardiographic assessment is essential in establishing the diagnosis of RV-D/RV-F [2, 31, 365, 375–378]. Vieillard-Baron [379] requires only the finding of RV-dilatation with a leftward shift of the septum in order to make a diagnosis of RV-D; however, there are many other echocardiographic features of RV-D/RV-F which can be used to confirm the diagnosis:
The RV is clearly dilated when the RV size ≥ LV size [358, 375, 376, 380]. The most common criteria with which to diagnose RV-dilatation is the RV/LV-ratio (assessed in the four-chamber view), but there is disagreement about the thresholds indicative of significant RV-dilatation, ranging from a ratio of 0.6–1.0 [381–383]; recent publications definitely assume the RV being dilated if RV basic diameter, measured in the 4-chamber-view at the RV base, exceeds 41 mm, or if the ratio RVEDD/LVEDD > 1.0 [2, 384].
The IVS becomes flat and bows towards the left ventricle in end-systole in case of predominantly pressure (over) load, thus, the right ventricle becomes circular at end-systole while the LV becomes eccentric in shape [31, 375, 376]. In end-diastole a countermotion is found [385]. This dyskinetic/paradoxical IVS movement, which is an effect of ventricular interdependence [2, 385], is indicative for RV pressure overload [2, 365, 384, 385]. Paradoxical septal movements may generally be a sign of an acute increase in RV-afterload [304]. In case of RV volume overload, a constant flattening of the IVS is seen leading to the so-called D shaped LV configuration [384, 385].
TAPSE shows a good inverse correlation to the pulmonary vascular resistance (TAPSE ~ 1/PVR) representing pulmonary hypertension in cases of elevated resistance [305]. TAPSE is afterload dependent and pathological values indicate an elevated RV-afterload [305]. It is an excellent measure of the systolic RV-function [387–389] as it has a direct correlation with RV-EF (TAPSE ~ RV-EF) [307, 309, 386, 388]. TAPSE is a highly sensitive and specific parameter of depressed RV-SV [390] as RV-SV indirectly correlates with PVR [391].
Additionally, a good correlation is established between the severity of the tricuspid regurgitation (TR) and TAPSE (TAPSE ~ 1/TR) [305]. A normal TAPSE value is >22 mm [305, 392, 393], while 15–19 mm excursion indicates a moderate depression of TAPSE [305] and when < 15 mm the outcome is very poor [305]. However, TAPSE < 17 mm indicates a RV-LV disproportion reflecting the series and interdependent (DVI) effects of the failing RV on the LV-filling [2, 170];
Hypokinesis of the free RV wall [365];
A TR-jet velocity of >2.8 m/s is suggestive of pulmonary hypertension [2];
Inferior vena-cava diameter (sub-costal view) > 21 mm during maximal) expiration (in spontaneously breathing patients) provides evidence for pathology [2]; if the amount of collapse is <50%, a pathologically high pressure is present, indicating pressure and/or volume (over)load [2, 394]. In mechanically ventilated patients the venous flow to the right heart is markedly reduced during inspiration secondary to the positive intrathoracic pressure reducing the amount of vena cava and hepatic vein collapse [395];
Furthermore, the newer Doppler-tissue imaging derived parameters such as tricuspid annulus S′ velocity or longitudinal strain of the free RV wall may be used for assessment [2, 384]
The pulmonary vascular resistance (PVR) may be calculated using echocardiographic parameters. PVR is calculated by the ratio of transpulmonary pressure (Δp) to transpulmonary flow (Qp):
PVR = Δp/Qp;
TR (maximal tricuspid regurgitant velocity) and TVIRVOT (time-velocity interval of the right ventricular outflow tract) can be used as a correlate to Δp (TR) and Qp (TVIRVOT) [396, 397]:
PVR = TR/TVIRVOT.
Abbas [308] found a very good correlation between PVRcath (measured invasively) and TR/TVIRVOT with a correlation coefficient r = 0.93, CI 0.87–0.96:
TR/TVIRVOT < 0.2 is most likely to be normal with PVR < 150 dyn × s × cm (80 dyn × s × cm−5 equals one Wood unit [400]):
The combination of a small and well contracting LV and a big, dilated and poorly contracting RV is pathognomonic for ‘acute’ right heart failure [401];
Interestingly, McConnell [304] has described severe hypokinesia of the mid free wall of the RV, but with a normally contracting apex, as pathognomonic of pulmonary embolism.
Features indicating possible de-compensation of RV-F are [15]:
Special clinical settings and their echocradiographic correlates [2]:
- (a)
Acute decompensation of chronic PH
• RV hypertrophy | • RV dilatation, spherical shape |
• Paradoxical septal movement, systolic/diastolic septal shift | • RA enlargement |
• Peak systolic velocity of tricuspid regurgitation >3.5 m/s |
- (b)
RV-AMI
• RV enlargement | • Global and/or regional hypokinesis |
• Abnormal septal motion | • TAPSE ↓ |
• Congested (dilated) V. cava (even if RV-pressures are normal or low) |
- (c)
Acute pulmonary embolism
• RVEDD/LVEDD ratio >1 (>0.9 [402]) | • McConnell’s sign |
• Tricuspid regurgitation velocities of 2.8–3.5 m/s | • Thrombi in the central pulm vessels |
• Systolic/diastolic spetal shifts: paradoxical septal movement; LV D-shaping |
4.4.5 Invasive Hemodynamic Assessments
Invasive hemodynamic assessments (and monitoring) are recommended in case the diagnosis is unclear or in therapy-resistant patients [2].
At rest, CVP normally equals 0 mmHg [403], and the CVP/RA-P are only elevated in disease states [404, 405]. Elevated (>8–10 mmHg) right atrial pressure/CVP is highly suggestive for acute right heart failure in a typical clinical setting [93]. A CVP ≥ 10–12 mmHg has already to be considered high, and will exert considerable constraint on LV filling [273, 278]. Thus, RA-P/CVP pressures ≥9–10 mmHg are always pathological and indicate that fluid application is highly unlikely to be successful [406] and that DVI will relevantly impact left ventricular filling, RV and LV filling pressures and the overall hemodynamic situation [273, 278].
4.5 Therapy
It has been emphasized that RV-afterload (PH) and altered myocardial perfusion/ischaemia are decisive factors in precipitating RV-F and the ability to therapeutically ameliorate these factors will determine the prognosis [1, 2, 27–30, 33, 135, 251, 284, 294, 301]. Thus, reduction of the elevated RV-afterload and avoidance or reversal of RCA-hypoperfusion are essential issues which therapy must address [1, 2, 133, 251, 407–410]:
Critical reduction of the increased RV-afterload
Avoidance/treatment of right ventricular myocardial hypoperfusion/ischaemia
Acute RV-F/acute exacerbation of RV-D/RV-F are reversible if the cause of the increased afterload can be treated [9, 33].
Furthermore, the hemodynamic consequences of RV-D/RV-F are the result of a
Other crucial targets are:
4.5.1 Specific Measures (Overview by [1–4, 9, 16, 20, 30, 33, 93, 98, 106, 107])
Specific treatment of broncho-pulmonary diseases
Treatment of systemic sepsis
ARDS: Therapy of underlying disease
Correction of valvular heart disease, and left heart failure
In acute myocardial infarction with involvement of the RV early reperfusion by primary PCI is essential [412–417]; read more about this issue in Chap. 3, cardiogenic shock.
Right heart dysfunction/failure and pulmonary embolism:
RV-F is the most common cause of death within 30 days following PE [110, 421] and RV dys-function is known to cause an increased mortality [110, 422, 423].
50% of all patients with pulmonary embolism present as clinically stable, without hypotension or circulatory failure, although suffering from RV-D [110, 365, 424]. They are at high risk of haemodynamic instability or even death during the first days after admission [425, 426].
The Shock Index is a sensitive parameter which can easily be used in daily practice in order to assess the potential outcome of patients with pulmonary embolism [289].


Thus, patients with a positive (≥1) shock index should be treated by thrombolysis (Evidence level A, Class I) [427–431].
Although not all studies give convincing evidence about the predictive and prognostic value of RV dysfunction [422, 423], Kucher [424] established that RV dysfunction is an independent prognostic predictor by analysing the data of the famous ICOPER study [110]. Patients with a systoli blood pressure ≥90 mm Hg (and thus classified as being hemodynamically stable/with preserved BP) but with RV-dysfunction had almost double the risk of death (16.3%) in comparison to those without RV-dysfunction (9.4%) over the first 30 days. Thus, although initially haemodynamically stable, all patients with RV dysfunction are at a high risk of death [424]. These results are consistent with those reported by Figulla [256], who found a 5–8% mortality rate in patients with normal BP but with RV dysfunction, while the prognosis of all patients without RV dysfunction was excellent (mortality rate 0–1%). It should be noted that the level of blood pressure taken as normal (sBP of >90 mm Hg versus >120 mm Hg respectively) was different in both studies and that the blood pressure on admission has a substantial impact on the patient’s prognosis [422] (see Table 4.2).
Table 4.2
Impact of blood pressure on patient’s prognosis
Clinical scenario | Mortality during hospital stay (%) |
---|---|
Normal BP, without RV-dysfunction | 0–1 |
Normal BP, with RV-dysfunction | 5–8 |
Hypotension, without signs of shock | 15 |
Hypotension and shock | Up to 35 |
Not all studies have concluded that thrombolytic therapy reduces the mortality significantly when administered to clinically stable patients with RV-D but preserved BP [422, 423]. Nevertheless, the haemodynamic situation clearly improved and stabilised immediately after the patients received thrombolytic agents [110, 423, 426, 432–434]. Furthermore, the first prospective study assessing the long term outcome after first-time ‘submassive’ pulmonary embolism in previously healthy patients treated by heparin and warfarin found 41% of the patients either with persistent or subsequently (weeks to months after PE) developed RV abnormalities or functional limitations [435]. The authors suggest that first-time pulmonary embolism is able to cause persistent right heart damage or to initiate a process which damages the RV over time. The main pathological mechanisms involved appear initially to be ischaemia of the RV subendocardium followed by an inflammatory response [303, 436, 437].
The results by Kucher [424], Figulla [422] and Woods [289] suggest that patients in shock and those with hypotension need thrombolytic treatment, but it would also seem more than wise—based on the current evidence—to consider patients with established proof of RV-dysfunction on an individual basis for thrombolysis as well.
More recent studies and trials still demonstrate roughly 7% hospital and 32% overall mortality in hemodynamically unstable patients with PE [438]. Even RV-D and elevated cardiac biomarkers are indicative for increased risk of in-hospital death and clinical deterioration [439]. All studies and metanalysis substantially support the application of thrombolytic therapy in hemodynamic unstable patients with massive PE (defined as hypotensive patients or patients presenting with syncope, cardiogenic shock, cardiac arrest, or respiratory failure due to acute PE [215, 420, 440]). On the contrary, hemodynamically stable patients with submassive PE (defined as patients with acute PE being normotensive but with signs of RV dysfunction [441]), there still is an ongoing controversial discussion whether a clinically significant benefit can be achieved by thrombolysis [440–442], even though those patients also suffer from an increased risk of early mortality and adverse outcome [441]: The largest study on systemic thrombolytic therapy in patients with submassive PE in fact revealed that thrombolysis in that condition is preventive for circulatory decompensations, but at the expense of an increased ratio of intracranial bleedings [440]. Marti et al. found in their metanalytic study a reduced overall mortality and PE recurrence rate, and further a reduction of PE associated death, if thrombolytic therapy was given to patients with acute PE. However, in hemodynamically stable patients the benefit was statistically insignificant. Moreover, thrombolysis in PE was in general associated with a considerable risk of major intracranial bleedings [420], and the mortality reduction found resulting from thrombolysis is basically offset by the risk of fatal, particularly intracranial bleedings in hemodynamically stable patients with submassive PE [420]. Thus in hemodynamically stable patients with submassive PE, initiation of thrombolysis has furthermore to be based on thoroughly individual evaluation.
4.5.2 Adjunctive Therapy [2, 9, 33, 358, 443]
4.5.2.1 Fluid Management and Optimization of Preload, Diuretics
The recommendations regarding fluid management in acute RV-D and RV-F have completely changed in recent years following a large amount of discussion [9, 193, 391, 443, 444]. RV filling above the physiological limit is accompanied by RV-dilatation [445].Thus, although some patients with RV-failure may respond to volume loading, fluid administration in acute right heart failure bears a high risk of further RV dilation/RV-chamber “overdistension” with its deleterious effects of increased RV wall stress, ensuing or worsening DVI, and reduced RV systolic power, diminished systolic LV support, the onset of tricuspid regurgitation or worsened TR, reduced LV filling and finally compromised CO and ischemia [446]. On its own, fluid administration in case of acute or acutely exacerbated right heart failure should basically be avoided because a beneficial effect of volume expansion can generally not be expected, even if there is a low LV-preload [193, 288, 290]. This is in particular the case if CVP exceeds 10–12 mmHg [406, 446]. Volume administration in this situation will not increase RV-SV and hence CO; in a depressed RV or in manifest RV-F only volume unloading will increase CO [287, 288]. Therefore, in the vast majority of patients suffering from acute RV-D/RV-F, volume loading has no benefit at all [9, 72, 168, 169, 193, 290, 391, 444].
On the contrary, diuretics are often the therapy of choice, since RV failure is usually associated with or even caused by RV volume overload, and diuretics may be safely applied in patients with venous congestion as long as the arterial blood pressures are maintained [2].
Diuretics are indicated in volume overloaded patients who have a dilated RV with leftward shifted septum and DVI following initial stabilization (maintenance of appropriate BP) of the circulation [348, 447]. Diuretics may induce metabolic alkalosis and thus aggravate hypoventilation and hypercapnia and, as such, should be used judiciously [448]. Moderate peripheral oedema should be tolerated in compensated chronic states [449, 450].
However, there are some exceptions to this rule. In the (few) cases of RV-F with normal PVR volume loading may be beneficial and increase preload, leading to an increase in RV-SV and LV-SV [444]. A well monitored (by CVP) and cautious volume loading may be further appropriate in case of systemic hypotension in the presence of normal right-sided filling pressures [2, 451–455]. Moreover, probably also patients suffering from acute myocardial infarction with significant involvement of the right ventricle are the group who will benefit most from controlled and balanced volume loading [251].
Ideally in daily practice, an echocardiogram to clarify the diagnosis, to assess the hemodynamic situation, and to guide therapy should be performed as soon as RV-D/RV-F and/or biventricular failure are suspected. However, as an emergency measure in shock or in haemodynamic instability [451–453, 456], as long as no clinical signs of fluid overload are present, a careful and well monitored fluid challenge is acknowledged to be always appropriate [451–455].
4.5.2.2 Vasopressors: Treatment and Avoidance of Ischaemia
Vasopressors directly increase the systemic blood pressure and thus improve the perfusion pressure of the RCA [301, 457–460]. Ghignone [461] and others [212] were first to establish that vasopressors may be the critical element in the treatment of acute right heart failure, as the administration of vasopressor drugs can break the pathological vicious cycle and avoid the manifestation of RV myocardial ischaemia [30, 212, 284, 294, 461].
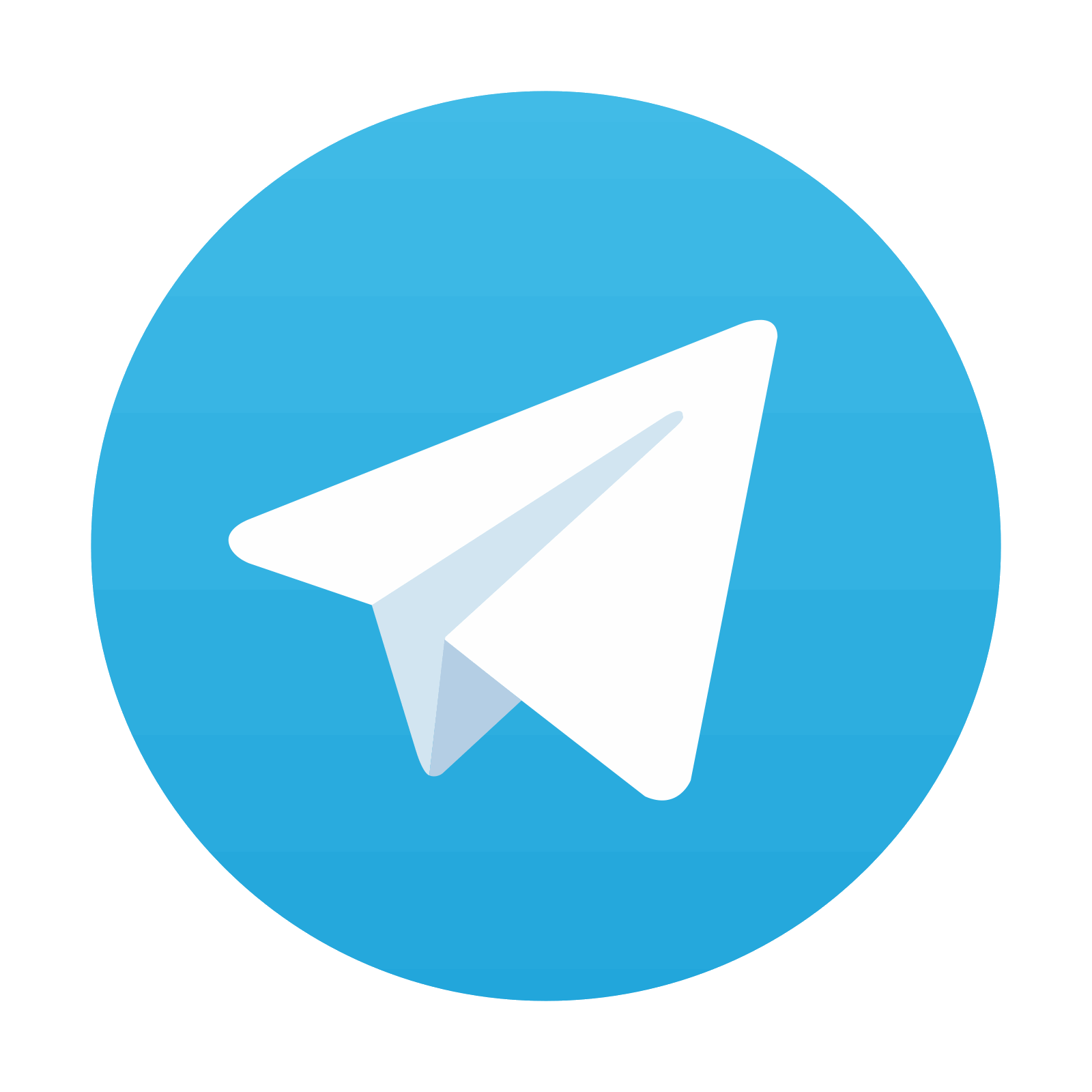
Stay updated, free articles. Join our Telegram channel
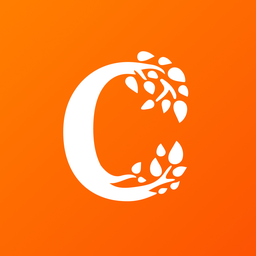
Full access? Get Clinical Tree
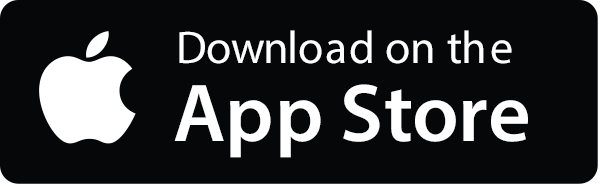
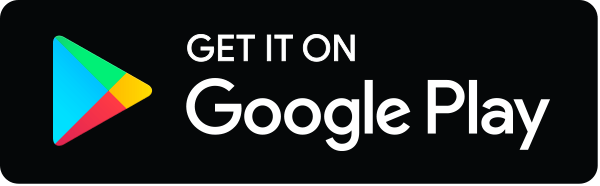
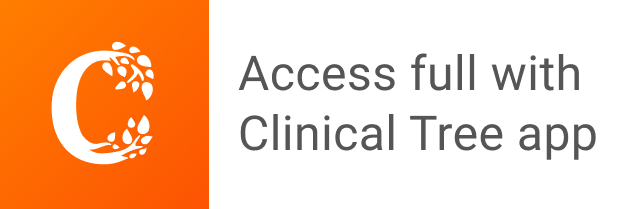