(1)
Kantonsspital Aarau, Medizinische Universitätsklinik, Aarau, Switzerland
Keywords
Pulmonary edemaNeurohormonal activationSystemic congestionPulmonary congestionHemodynamic congestionClinical congestionInflammationEndothelial dysfunctionNO bioavailabilityVentriculo-arterial stiffeningCardiac performanceVacular toneVascular stiffnessVascular failureCardiac/cardio-renal failureRedistributionFluid accumulationVenous capacitanceBlood pressure (BP)Hemodynamic profilesDiureticsVasodilatorsInotropic drugs2.1 Definition
As yet, no definition of heart failure is universally accepted, however, heart failure may be defined as “the inability of the heart to supply the bodies’ tissues sufficiently and suitably with blood meeting their metabolic demand or do so only at the cost of elevated filling pressures” [1–3].
The European Society of Cardiology (ESC) defined acute heart failure in 2005 as “the rapid onset of symptoms and signs secondary to abnormal cardiac function. It may be occur with and without previous cardiac disease” [4]. In their 2012 guidelines, the ESC modifies and states heart failure to be subject to “an abnormality of cardiac structure or function leading to failure of the heart to deliver oxygen at a rate commensurate with the requirements of the metabolizing tissues, despite normal filling pressures (or only at the expense of increased filling pressures)” [5], confirming a definition developed by a joint expert group consisting of the ESC Heart Failure Working Group and the European Society of Intensive Care Medicine (ESICM) [6].
The ACCF/AHA Practice Guideline from 2013 defines, “heart failure is a complex clinical syndrome that results from any structural or functional impairment of ventricular filling or ejection of blood” [7].
2.2 Classification of Acute Heart Failure Syndromes (AHFS)
Acute heart failure may occur as an acute de novo event without previously known cardiac malfunction or as an acute decompensation of chronic heart failure [4].
The ESC Task Force Group has classified acute heart failure into six distinct pictures. This is based on the clinical condition at presentation and the hemodynamic characteristics described by Forrester [11], Killipp [12] and more recently by Cotter [13], along with a report and explanation by Adams [14] and in accordance with a publication by Gheorghiade [15]. This, in 2005 introduced classification, is still widely used [16–20], although some authors replaced high output failure, ESC-5 (due to its imprecise specification with the various underlying entities, ahead of all septic shock), by acute heart failure complicating acute coronary syndrome (ACS) which requires a especial treatment (immediate coronary angiography and intervention) [19, 21].
Classification based on Nieminen [4] and Gheorghiade [15], modified and replenished by Joseph [16].
ESC-1: Acute Decompensated Heart Failure (AD-HF)
De novo or decompensated chronic HF.
Signs and symptoms of acute HF are generally mild and do not fullfil criteria for cardiogenic shock (CS), pulmonary oedema, or hypertensive crisis (HTN).The onset is gradual, peripheral edema often significant, while pulmonary congestion may be really discrete.
ESC-2: Hypertensive Acute Heart Failure (hypertensive AHF)
Characteristic signs and symptoms of HF are accompanied by high blood pressure (BP) and a chest radiograph which is consistent with acute pulmonary congestion, while left-ventricular systolic function is relatively preserved or even normal. Often rapid onset, marked dyspnea, altered mental status, and oliguria/anuria are possible (Table 2.1).
Table 2.1
Hemodynamic profiles
ESC-1 | ESC-2 | ESC-3 | ESC-4 | ESC-5 | ESC-6 | |
---|---|---|---|---|---|---|
Heart rate | = | ↑ | ↑ | ↑ | ↑ | ↓/↑ |
Systolic BP | N/↑/↓ | ↑/↑ ↑ ↑ | Low N/↑ | N/↓–↓ ↓ ↓ | N/↓/↑ | ↓/↓ ↓ |
Cardiac index [l/min/m²] | Low N/↓/↑ | N/↑/↓ | ↓ | <1.8–2.2–↓ ↓ ↓ | ↓↓/N | <2.2/↓↓ |
PCWP [mmHg] | ↑, ≥12–16 | ↑, >18 | ↑, >16 | ↑↑, >16–18 | N/↑↑ | ↓, <12 |
Congestion | +/+ + | +/+ + + | + + + | +/+ + | −/++ | None |
Urine output | −/+ | −/+ | + | Low/None | +/− | Low/None |
End organ hypoperfusion | −/+ | −/+ | −/+ | + +/+ + + | −/+ + | −/+ |
II | II–III | II/IV | III/IV | I–II | I–III |
ESC-3: Pulmonary oedema
Symptoms and signs compatible with pulmonary oedema, normally accompanied by severe respiratory distress with SaO2 usually <90% on room air prior to treatment, and a chest X-ray showing pulmonary oedema.
ESC-4: Cardiogenic Shock (CS)
The patient exhibits evidence of tissue hypoperfusion induced by HF although pre-load is appropriate or has been properly corrected. There is no clear definition of hemodynamic parameters, but CS is usually characterized by reduced BP (systolic BP < 90 mmHg or a drop of mean arterial pressure of >30 mmHg), and/or low urine output (<0.5 mL/kg/h) with a pulse rate of >60/min, with or without evidence of organ congestion.
There is a continuum from low cardiac output syndrome (hypoperfusion, oliguria, and hemodynamically a low normal sBP, a CI < 2.2 L/min/m2, and a PCWP >16–18 mmHg) to CS (marked hypoperfusion, oliguria/anuria, hemodynamically a sBP < 90 mmHg, a CI < 1.8 L/min/m2, and a PCWP > 18 mmHg).
ESC-5: AHF complicating acute coronary syndrome (ACS) (has replaced high output failure)
May clinically impress with pulmonary edema (ESC-3), pre-shock or manifest shock or as a cold and dry type (ESC-6). Life saving measure is immediate angiography and revascularization (evidence level A, class I recommendation) [22–26].
ESC-6: Right Heart Failure (RV-HF)
Characterized as low output syndrome with ↑ jugular venous pressure, increased liver size, and hypotension, often poor perfusion, but clear lungs.
2.3 Aetiology and Epidemiology [4, 14, 27–30]
The main causes of acute heart failure syndromes are:
Coronary (ischaemic) heart disease/ischemic cardiomyopathy;
Valvular heart disease;
Dilated cardiomyopathy;
Hypertension/hypertensive crisis and hypertrophic cardiomyopathy;
Acute arrhythmias;
Acute endocarditis;
Restrictive cardiomyopathy;
Acute pericarditis/cardiac tamponade;
Acute (peri) myocarditis;
Aortic dissection;
Extracardiac diseases:
Broncho-pulmonary diseases, particularly those producing hypoxic states, e.g., acute exacerbation of COPD or severe pneumonia;
Anaemia;
Hyper/hypothyroidism, and other endocrine diseases;
Fluid overload;
Drug-induced heart failure;
Metabolic/toxic reasons;
Infectious diseases (particularly sepsis as high output heart failure);
Neuromuscular diseases such as the myopathies;
Trauma.
Coronary artery disease (CAD) is the underlying cause of heart failure syndromes in the majority of cases [16, 26, 31]. Rudiger [12] conducted a European survey showing that CAD was the underlying disease in 62% of cases. Other studies have confirmed this result showing CAD as the main aetiology of acute heart failure in 60–70% of all cases [28, 29, 31, 32]. Valvular heart disease is reported in up to 44% (seems very high!), dilated cardiomyopathy is prevalent in 25% [33].
Up to 70% of all heart failure patients admitted, suffer from arterial hypertension [14, 28], diabetes mellitus is found in 40%, and impaired kidney function was present in 20–30% [16, 31]. Atrial fibrillation/atrial flutter is seen in 30–40% of patients [16, 31].
The vast majority of all patients admitted with acute heart failure (approximately 75% [14, 16]) suffer from an acute decompensation of chronic heart failure, often decompensated due to systemic infection, treatment with cardio-depressive drugs, reduction of the patient’s cardio-specific medication, pulmonary embolism, or inappropriate physical stress [14, 28]. About 50% of all AHFS suffer from HFpEF [14, 34–36].
The main reason for acute HF in patients with ‘preserved systolic’ function, HFpEF, (EF > 50%) [37–39]) is an acute increase in systolic blood pressure [40, 41], but new onset of atrial fibrillation (AF) is a frequent reason as well [42].
Less than 10% have advanced heart failure [16].
Acute heart failure is the discharge diagnosis in about one million patients of all ages each year [43]. The overall in-hospital mortality is as high as 5% [44], the 30-day one is 10–12% [45]. 33% will die within the first year following their first admission [46]. The 5-year mortality rate remains high, around 50% [47, 48].
The prognosis may be even worse as a recently published survey by Zinnad [49] revealed: In contrary to other surveys, this French survey included not only patients suffering from acute heart failure admitted to general and cardiology wards, but also severely ill patients requiring CCU or ITU admission. The number of patients with pulmonary oedema (82%) and cardiogenic shock (29%) was substantially higher than reported in previous studies [28–30]. The mortality in this study was as high as 27% at 4 weeks and 62.5% after 1 year.
2.4 Pathophysiology
2.4.1 General Remarks
Since the majority of patients admitted to an emergency department with heart failure display acutely worsened heart failure symptoms, acute heart failure (AHF) has recently been referred to as “an increase in the severity of chronic heart failure symptoms that requires an escalation of therapy and hospitalization” [50].
Both, acutely decompensated chronic heart failure and newly arisen, “de novo” cases without prior history are perceived as AHF, respectively known as acute decompensated heart failure (ADHF) or acute heart failure syndromes (AHFS) [6, 51, 52].
The pathogenesis of acute heart failure syndromes is complex and of multifactorial origin, however it is basically attributed to the interplay and interconnection between essentially cardiac disorders (e.g. altered diastolic and/or systolic features including ischemic or hypertrophic cardiomyopathies) with systemic afflictions, mainly altered vascular properties affecting loading conditions (as arising in hypertension, inflammation and infections, metabolic maladies, and as a consequence of modifications of adaptive measures in neuro-endocrine activity (NH)) [5, 7, 17, 50, 53, 54].
Changes in total body fluid content (fluid retention and accumulation) and fluid shifts within the body’s compartments (central fluid redistribution) [53, 54], impacting LV and RV preload, are essential elements of the pathogenetic processes [53, 54]. Furthermore, typical comorbidities frequently seen in heart failure patients including pulmonary maladies like COPD, sleep-distorted breathing disorders, and renal dysfunction/worsening renal function, directly influencing cardiac and myocardial characteristics and likewise afterloading conditions [53], are well embedded in the pathobiology [5, 7, 17, 53, 54].
Typical, classic features precipitating acute decompensations and being responsible for the AHF incidence in more than 80% of all cases include:
Ischemia/acute coronary syndromes;
Systemic infections, notably respiratory tractus infections;
Poorly controlled co-morbidities, such as exacerbated COPD with and without pneumonia;
Uncontrolled hypertension/acute hypertensive dysregulations;
Arrhythmias (atrial/ventricular arrhythmias);
Nonadherence to medication;
Renal failure/worsening renal function;
Nonadherence to diet/inappropriate salt intake;
Inappropriate physical stress
Some special notes:
Ischemia: Ischemic injury diminishes LV compliance, hence causes an increase in ventricular stiffness [61], subsequently LVEDP rises [62, 63]. Backward transmission of the elevated filling pressures puts the lung at risk for congestion or even pulmonary edema [64–67].
Furthermore, any rise in filling pressures bears the risk for (further) ischemia, as elevated intra-cavitary pressures may compromise endocardial perfusion, particularly in cases of coronary artery disease and already poor perfusion pressure as in hypotensive states [66, 68].
Around 60% of patients with ADHF definitely suffer from coronary artery disease [69], while myocardial ischemic events are more common in de novo AHF [26, 33].
Comorbidities such as obesity, diabetes, hypertension and COPD promote inflammation, moreover they may be seen as low grade inflammatory maladies [70, 71]. Increased levels of inflammation are independently associated with asymptomatic diastolic dysfunction [72], and all these maladies have been verified to be independently associated with early development of diastolic LV dysfunction [73–75]. Moreover, systemic inflammatory conditions are predictive of incident HFpEF [70]. As such, by affecting diastolic function leading to increases in filling pressures [41, 76–79], these comorbidities are acknowledged to decisively contribute to or even cause heart failure [80]. Acute worsening of diastolic properties are well recognized to precipitate AHF [81, 82]. Read more on this issue in Chap. 5, HFpEF !
Arrhythmias: Atrial fibrillation or flutter (AF) is prevalent in 30–45% of patients admitted with ADHF [83–85]. AF in the presence of AHF is associated with a worsened prognosis and an increased rate of mortality [86, 87]. Both, systolic and diastolic dysfunction are associated with the risk for incipient AF [88]. Atrial fibrillation/flutter and heart failure interact in a deleterious way and fast heart rates due to AF may initiate de novo AHF, or may substantially worsen chronic heart failure [89, 90]. AF affects relevantly hemodynamics and left ventricular function [91] due to the loss of both atrial contraction, which is essential for sufficient ventricular filling, and the tachycardia itself [92]. Thus, diastolic filling is limited due to the high heart rate and the loss of atrial contraction, and that, in the presence of an already compromised cardiac performance, is one of the mechanisms able to cause ADHF [93]. Furthermore, the tachycardic heart rate may display tachycardia-induced cardiomyopathic effects [94]. Accompanying further activation/accentuation of the already stimulated (as in chronic heart failure) NH contributes to the detrimental events [95]. Patients with diastolic dysfunction and HFpEF are especially affected due to their subjection on a proper atrial contraction to assure a suitable LV filling [94].
Renal dysfunction/worsening renal function: A cross-talk between the heart and the kidneys, affecting function and performance of each other, is well established [96–98]. In the setting of heart failure, venous congestion, neurohormonal activity, inflammation as well as endothelial dysfunction are the main trigger and contributors to baseline renal dysfunction, by altering intrarenal and intraglomerular hemodynamics [97, 99, 100]. However, in contrast to traditional views, venous congestion rather than compromised cardiac output decisively contributes to worsening renal function (WRF) in most cases [101, 102].
Elevated filling pressures, enhanced peripheral resistance (more precisely increased aortic input impedance which best reflects and represents systemic afterload to cardiac pump as a whole [103]), diminished natriuresis, and often (generally in case of HFrEF), but by far not always, a reduced CO are the hemodynamic hallmarks of heart failure [5, 6, 104, 105].
However, the clinical picture presented by patients suffering from AHF always look very alike and is characterized by signs and symptoms related to pulmonary and peripheral congestion, regardless whether suffering from HFrEF or HFpEF [5, 17, 50, 106]. Bedside physical examination, lab-tests, and X-ray are not able to distinguish between both entities [106, 107]. However, history and response to therapy provide considerable clues as to which type is probably underlying: HFpEF patients are generally older, are significantly more likely to be obese and have a high(er) BMI, moreover, 85% suffer from the metabolic syndrome, hypertension and AF are considerably more often seen in HFpEF than in HFrEF, and HFpEF patients less often have a history of coronary and valvular heart disease [47, 108, 109]. Iron deficiency is more often found in HFpEF [109]. BNP levels are generally significantly elevated and higher in decompensated HFrEF compared to those demonstrated in HFpEF, moreover, 1/3 of HFpEF patients do not show noteworthy elevated BNP levels at all, although acutely decompensated [110, 111]. This circumstance is explained by the lower end-diastolic wall stress triggering production and release of BNP, emerging in HFpEF due to remodelling processes, e.g. hypertrophy [110].
Since patients with HFpEF are considerably sensitive to changes in BP, their pressure may significantly fall in case of application of diuretics or vasodilators [112, 113], a phenomenon not found in HFrEF patients who generally benefit symptomatically from administering vasodilators or diuretics as long as a sufficient BP (systolic ≥100–120 mmHg) is measured before these are given [112].
2.4.2 Special Pathophysiological Issues
2.4.2.1 LVEDP and Congestion
The main factor and source causing AHF symptoms is congestion rather than low CO [14, 28, 114, 115]. Only a minority of patients (clearly less than 10%) admitted with AHF feature signs and symptoms of clinically relevant, significantly compromised peripheral circulation, hypoperfusion, and/or clinically meaningful hypotension [116–118]. As such, both entities (HFpEF and HFrEF) share one of the main pathophysiological issues indicating and promoting acute heart failure: acutely and substantially elevated left, and generally subsequent right [15, 119–121], ventricular filling pressures, which are associated with pulmonary and systemic venous congestion (with and without low CO) [14, 15, 122–124]. Left- and right-sided filling pressure is largely determined by (a) the amount of blood flow (venous return) to the heart and (b) by the diastolic cardiac properties, e.g. chamber and myocardial stiffness [125]. Accordingly, a considerable high blood volume flow to the heart (preload) alone may precipitate pulmonary edema as seen in completely normal hearts of patients suffering from acute glomerulonephritis, causing acute, oligo/anuric kidney disease [125]. On the other hand, worsening diastolic dysfunction (DD) is shown to provoke pulmonary edema even in the absence of relevant volume retention [113, 126, 127].
Backward transmission of the elevated left-sided filling pressures, causing pulmonary venous hypertension augmenting RV afterload [4, 128–130] and diastolic ventricular interaction [131, 132], decisively affect and, in turn, lead to marked increases in RVEDPs consecutively displaying systemic (peripheral) congestion [133, 134]. As such, congestion attributed to high LVEDP is responsible for and causes the foremost clinical symptoms, dyspnoea (acute dyspnoea at rest, orthopnoea or paroxysmal nocturnal dyspnoea, breathlessness on exertion), and signs and symptoms associated with peripheral edema development, like swollen legs, ascites, renal dysfunction and gut discomfort [17, 30, 49, 50, 69]. Considerable evidence indicates that elevated, high LVEDPs causally underlie the development and presence of congestion [123]. Moreover, every relevant acute rise in LVEDP may precipitate pulmonary congestion or even flash pulmonary edema [67, 135]. Patients suffering from diastolic dysfunction are particularly at risk to develop pulmonary congestion or edema as any (additional) pathological effect affecting the heart muscle potentially worsens diastolic stiffness [126]. Typical conditions are acute ischemic episodes [136] and abrupt increases in BP [4, 113, 127, 137]. Ischemia causes (further) ventricular diastolic stiffening [138, 139] as acute myocardial ischemia slows ventricular relaxation and increases myocardial wall stiffness [140–142], consequently LVEDP increases [62, 63, 142]. Rising BP is, in any case, associated with elevated sympathetic tone, augmented afterload, increases in LVEDP, and may result in fluid redistribution and further neurohormonal activation [15, 143, 144]. Increases in afterload generally cause a rise in LVEDP [113, 127, 145, 146]. As such, HFpEF patients, found to be highly sensitive to changes in loading conditions (volume and pressure load) [113, 147, 148], are especially predisposed to develop pulmonary congestion or actually flash pulmonary edema [67, 113, 127, 149]. This is even true in the case of only mild, acute increases in BP [67, 113, 127, 149] or yet undetectable volume expansions [148]. In a rigid heart chamber, which is unable to properly accommodate to increasing blood flows and intracardiac volumes [150], already small increases in end-diastolic filling volume are accompanied by substantial, exponential (the diastolic pressure-volume relationship follows an exponential equation) increases in LVEDP [150]. Indeed, rising and elevated BPs or increasing LV filling volumes may lead, in the setting of combined ventriculo-arterial stiffening, to further increases in ventricular stiffness [113, 148, 151], thereby worsen diastolic dysfunction [152] which will result in disproportionate rises in LVEDP [113, 148]. Worsening diastolic function due to hypertensive dysregulations, uncontrolled hypertension, and myocardial ischemia, but hyperglycemia as well, are predominant causes for AHF development in HFpEF patients and in diabetic patients with diabetic cardiomyopathy [40, 41, 152]. However, new onset of atrial fibrillation (AF) is, as well, a frequent trigger [42]. As a result of the loss of atrial function, a compensatory increase in LVEDP in order to maintain end-diastolic filling volume and thus CO (via Frank-Starling mechanism) is reported. Subsequently the neurohormonal systems will be activated [152]. Both, reduced diastolic filling and abnormal left atrial function, may result in neurohormonal stimulation [152]. Increases in LVEDP are demonstrated to happen more rapidly in HFpEF than in HFrEF, attributed to the blunted diastolic distensibility, a typical property of HFpEF (in contrary, HFrEF patients show an increased diastolic distensibility) [123].
Accordingly, elevated LVEDPs causing central, pulmonary and systemic congestion are in the vast majority of AHFS the critical underlying pathology and the reason for presentation [5, 122, 153, 154]. Remarkably, central and peripheral congestion usually arise concurrently [155, 156].
Enhanced levels of left ventricular filling pressures unfortunately display a range of adverse effects, including enhanced myocardial oxygen demand, compromised coronary perfusion with concomitant risk of angina, global and subendocardial ischemia [157–159], progressive mitral [15] and often tricuspid regurgitation, activation of the adrenergic and the renin-angiotensin-aldosterone system, thus activating the neurohormonal systems (NHs), and stimulate the cytokine system [160]. As such, high LVEDPs may evoke and contribute to disease progression [104, 161–164]. Indeed, features typically associated with and characteristic of AHF are neurohormonal activation [165–168], stimulated inflammation [169–172] and activated endothelial function, commonly termed endothelial dysfunction (ED) [173, 174]. Inflammation and ED generally accompany each other as they are closely interrelated and interconnected [175–177] and go along with enhanced levels of oxidative stress (ROS) [178, 179].
It should be noted that acute severe left heat failure may occasionally, in individual cases, not be accompanied by high filling pressures (markedly dilated ventricles, often with severely impaired systolic function) featuring a normal or even low LVEDP and no pulmonary edema, although they do suffer from severe acute left heart failure [180–182]—this is the so-called ! “forward failure” as described by the ESC [4].
2.4.2.2 Neurohormonal Systems, Endothelial Dysfunction and Inflammation
The neurohormonal systems, NHs, perform necessary and pivotal control and modulating functions and exert a substantial integrative impact on cardio-circulatory physiology [183–185]. Neuro-hormones carry hemodynamic and biological effects on the heart and the vascular system [186]: Augmented sympathetic discharge is not only exerting vasoconstrictive, positive inotropic and chronotropic effects, but is going along with blunted parasympathetic drive causing abnormal cardiopulmonary reflex control, including attenuated baroreflex and boosted peripheral and central chemoreflexes [168]. As such, autonomic imbalance (excess sympathetic discharge and coexisting withdrawal of parasympathetic tone) is a characteristic feature in heart failure [187]. Stimulated renin-angiotensin-aldosterone system [165] and augmented non-osmotic release of arginine-vasopressin, promote other than systemic and local vasoconstrictive effects, especially renal functional changes, namely declined ultrafiltration and retained sodium and H2O, thus facilitate fluid accumulation [166, 186]. Endothelin-1 causes marked vasoconstriction (enhancing vascular tone) [188], while elevated concentrations of natriuretic peptides especially counteract the vasoconstrictive (show venodilative and peripheral arterial resistance lowering effects) and the fluid retaining effects of the NH mediators and hormones [167, 189–191]. However, their ameliorating effects on the sympathetic and RAAS discharge appear to be clinically of minor potency in acute heart failure [164, 192], and their clinical importance is attached to their diagnostic and prognostic power [193–195]. Of special note, elevated A II and aldosterone levels contribute (aside from their well-known and characteristic vasoconstrictive effects, which directly augment the systemic vascular resistance and as such the afterload [196], and their sodium and water retaining impact [197–199]), through endothelial activation and enhanced ROS generation,1 to the considerably diminished NO bioavailability [202–206], typically emerging in AHF [174, 206]. As such, A II promotes endothelial dysfunction and augmented ROS generation, and thereby a markedly diminished NO bioavailability ensues [161, 202, 206]. This results in significantly impaired endothelial NO dependent vasodilatation causing increased vascular tone (vasoconstriction) and disturbed regulation of ventricular function: “NO dependent regulation of ventricular function and vascular tone determines hemodynamics in AHF” [174]. The important NO-cGMP-PKG signalling pathway (NO is a pivotal paracrine and autocrine signalling molecule [207]), is a universal cascade of cellular communication regulating, via protein phosphorylation, gene expressions [208–210]. This signalling pathway will be affected resulting in (a) altered smooth muscle cell relaxation which concomitantly impacts local and systemic blood flows and blood pressure [211], causing vascular dysfunction, and (b), related to an afflicted cardiac endothelium, disturbed titin phosphorylation within the cardiomyocytes [208–210]. Titin hypophosphorylation leads to cardiomyocyte stiffening and thus precipitates diastolic dysfunction [78, 112, 210, 212]. Hence, vascular compliance (namely of the central larger vessels) is diminished (vasoconstriction in arteriolar vessels reduces arterial compliance [213]) causing increased vascular stiffness and subsequently enhanced LV (RV)-afterload thereby also facilitating diastolic dysfunction [147, 148, 174, 214–216]. Furthermore, a rise in systolic ventricular elastance is induced and as such augmented ventricular (end-)systolic stiffness [214, 217] impairing systolic performance/reducing systolic reserve capacity [199, 218]. Indeed, augmented arterial stiffness is associated with both, systolic and diastolic dysfunction [219–221], and moreover, considerably impaired NO bioavailability and worsening endothelial dysfunction are even suggested to propagate the development and/or progression of heart failure [175, 222, 223].
Without doubt, in the early phase of AHF, enhanced neurohormonal activity allows stabilization of the compromised hemodynamic conditions and disrupted homeostasis jeopardizing suitable tissue and organ nutrient and oxygen supply [224]. Ventricular filling pressures increase with increasing sympathetic tone [225] and thereby may assure appropriate ventricular filling volume in order to maintain CO in the failing heart. On the other hand, they may contribute and provoke pulmonary congestion or edema [226]. Over time, the effects of the NHs, if persistent and chronically activated, are considered and appraised to be maladaptive, deleterious for the circulation, and leading to disease progression [54, 118, 164, 192, 224, 227].
Moreover, very recent publications suggest that the stimulated neuro-endocrine hormonal systems are even “over-activated” and are overwhelming the counter-regulatory cascades, decisively contributing to, mediating, and maybe even precipitating acute heart failure [60, 162–164, 183, 186, 227, 228] as they considerably modulate myocardio-mechanical properties [229].
Endothelial activation (EA)/dysfunction (ED) being present in AHF is evidenced by elevated levels of biomarkers indicative for EA including vascular adhesion molecules (VCAM-1) and intercellular adhesion molecules ICAM-1 [230–232], cytokines such as IL-6 and IL-1β, and tumor necrosis factor TNFα [233–235]. ED is meanwhile acknowledged taking a central and crucial role in the pathophysiology and pathogenesis of acute and chronic heart failure [175, 223]. Endothelial factors and mediators, whereupon endothelial relaxing factor (NO) activity represents a hallmark of endothelial function [236], contribute via para-, auto- and endocrine pathways to organize pivotal homeostasis and co-modulate cardiac and renal assignments and vascular properties in order to assure appropriate blood volume, cardiac output, perfusion pressure and blood distribution to the tissues and organs meeting cellular and tissue metabolic demands [192, 236].
Altered endothelial function, endothelial dysfunction (“should more appropriately considered as endothelial activation” [237]), implies a disturbed NO bioavailability, among other issues (ED displays pro-inflammatory, pro-coagulatory, and vasoconstrictive conditions [238]), as probably its most relevant pathobiological consequence. A disturbed NO bioavailability critically contributes to an imbalance of local (and potentially systemic as the endothelium is present in the whole body) vasoactive substances [239–241], precipitating significantly increased vascular tone, resulting in deranged (local) blood flow distribution and autoregulation [242]. The restricted bioavailability of NO is not only associated with vasoconstriction but causes increased stiffness in the systemic and pulmonary circulation and hence augments LV and RV systolic load [174]. Shortage of NO availability further favours ET-1 related vasoconstriction [243], increases sympathetic discharge including raised release of catecholamines [244], and contributes to diminished sodium excretion [245]. Dysfunctional cardiac microvascular endothelium may affect, via paracrine paths, diastolic LV properties [206] whereupon the already mentioned NO signalling pathway, considerably affected by ED, precipitates compromised endothelial cross-talk and disrupted phosphorylation paths [246, 247], including the cardiomyocyte NO-cGMP-PKG pathway leading to titin hypophosphorylation and thus acute cardiomyocyte stiffening [210, 218]. Accordingly, the effects of ED relevantly contribute to the clinical-hemodynamic profile characteristic in heart failure [248]. Moreover, ED is related to heart failure initiation and thus AHF [249]. ED is associated with adverse outcome in acute and chronic heart failure [250–252], correspondingly improvement of endothelial function is affiliated with a better outcome [253]. The worse ED the more severe the heart failure stage present and the more severe the functional limitation [254, 255]. ED independently predicts mortality risk [249, 251, 256] and major cardio-vascular events [252]. Hence, there is no doubt that ED has a major and crucial role in heart failure malady in both acute and chronic conditions, integrates the multi-facet signals arising [176], triggers, modulates and even perpetuates the cascades activated [257–259]. Indeed it orchestrates the adaptive and potentially morbid processes, and unquestionably causally contributes to initiate and to display acute heart failure [174, 260]. Notably, dysfunctional vascular endothelium is a recognized hallmark of human diseases in general [261].
Inflammation as evidenced by elevated serum levels of TNFα, IL-1, IL-6, and ST-2, an activated complement system and adhesion molecules verified in AHFS is part of the pathobiology [170, 171]. Inflammation per se is a protective response to injury of any kind, ensues and applies by interactions between cell surfaces, extracellular matrix, and pro-inflammatory mediators [262], and may basically be regarded as a vascular response to any threat or injury [263–265]. As such, vascular stretch as present in acute and chronic pressure or volume load exerts biomechanical stress on the mechanoreceptors of the endothelial cells, and thus initiates an at least mild inflammatory response [266–268]. Even physiological adaptions in vascular tone and tension are principally regulated and mediated by the same molecules, agents, and hormones involved in inflammatory processes [17] and in endothelial functions and effects. The endothelium is substantially involved in the innate [269–271] and adaptive [272]) immune response to injury, processes associated with inflammation, and as such is surely a fundamental feature in cardiovascular disease processes and may be considered as “linking” inflammation and cardiovascular diseases [174, 175, 206]. Indeed, a distinct correlation between inflammation and endothelial dysfunction is well established [273], confirming that inflammation and endothelial cells are closely intertwined and interconnected [273, 274]. Furthermore, since being characterized and accompanied by an increase in inflammatory markers including CRP, cytokines, adhesion molecules, and acute phase proteins, a more substantial and chronic inflammation has to be considered as a systemic process rather than “purely” a local reaction [275]. Accordingly, inflammation is recognized as taking a central position in the pathophysiology of cardiovascular diseases and hypertension [276]. As such, inflammation may, by causing endothelial dysfunction [277, 278], promote hypertension due to impaired endothelium-dependent vasodilation favouring vasoconstriction following an imbalance between vasoconstrictor and vasodilator mediator production and release [279], namely an impaired NO bioavailability [280, 281].
Especially remarkable and important to understand is that systemic inflammation such as severe infections, especially sepsis, may cause acute cardiac decompensations: The set of cardio-vascular responses associated with inflammatory activation may include a dissociated reply with enhanced peripheral vasodilation (due to reduced peripheral vascular resistance caused by vasodilative mediators). Although occurring in the presence of limited NO bioavailability, which implicates impaired NO-related vasodilation, and coexisting with enhanced arterial stiffness, which consecutively causes increased afterload [282, 283], the net result is a potential drop in blood pressure (largely determined by the resistance vessels), LV afterload is augmented [174, 219, 284, 285] and systolic LV-function is blunted [174, 219, 286].
To resume this issue, beside the tight and intertwined relation between ED and inflammation [273, 274], a close interrelation and interaction between the NHs, namely the autonomic nervous system and the AII effects, and endothelial function/dysfunction is quite evident [287–289], whereupon NO constitutes the decisive link [272]. Enhanced sympathetic drive induces shear stress on the vessel walls [290], even in case of minor discharge [291]. Shear stress is associated with ED [292–294]. On the other hand, (activated) endothelial cells may trigger NHs [287, 295]: Physiological and pathological (as e.g. in case of venous congestion) biomechanical forces affect the endothelial mechanoreceptors and subsequently stimulate endothelial cells and consecutively activate NHs [201, 261, 296]. Endothelial stretch (activating endothelial cells) [201, 261, 297], and as such even increasing ventricular filling pressures and myocardial stretch [160], directly evoke activation of the NHs, in case of SNS by altered autonomic reflexes [297]. Actually, elevated filling pressures and myocardial stretch are acknowledged as being among the most powerful impulses activating NHs [160]. Accordingly, NHs activation and endothelial dysfunction may be considered being a common path of the causative features contributing to incipient heart failure [186]. Furthermore, the tight interrelations and interconnections of the features and systems inevitably hold the risk to end up and to constitute a vicious cycle, potentially amplifying and perpetuating each other and as such facilitating disease progression [60, 104, 259, 297, 298].
2.4.2.3 Vascular Properties, AV-Coupling, Afterload Mismatch and the Dual Pathway Concept by Cotter
Vascular properties and “function play a central role in the development and progression of heart failure” [174]. Albeit the elevated left-sided (and mostly right-sided as well) filling pressures, which are associated with central pulmonary and peripheral congestion [14, 15, 122, 123, 153], coin the clinical picture and give rise for hospital admission in the vast majority of acute heart failure cases [17, 30, 49, 50, 69], acute marked alterations in vascular tone, vasoconstrictions (affecting LV and RV loading conditions) are actually often the direct cause of incipient AHF [13, 113, 127, 137]. Indeed, AHF may be considered as a disorder of “pathologic vasoconstriction” [299]. AHF is almost always associated with both, elevated LVEDPs and generally (in cardiogenic shock as the most severe form of AHF, the SVR may be even abased – read more about this phenomenon in Chap. 3) substantially increased afterload, in daily practice usually indicated by augmented peripheral vascular resistance (SVR), whereupon CI/CO is indefinite [300].
The often considerable increase in afterload (respectively SVR as part of total afterload) in the presence of deficient systolic and/or diastolic myocardial properties [300] cannot be compensated, as the cardiac properties are neither capable of allowing compensation by increasing preload (at least not at the cost of still reasonable increases in filling pressures below the threshold of pulmonary congestion/edema formation) and consecutively applied Frank-Starling-mechanism nor by subsequent sufficient increase in contractility following and adapting to the rapid increase in aortic pressure (afterload), known and referred to as Anrep’s effect [301, 302]): This condition (a substantial rise in afterload in the presence of compromised systolic and/or diastolic capabilities) is referred to as afterload mismatch [303, 304]. Afterload mismatch causes a vicious cycle with secondary mitral regurgitation, reduced SV and increasing and elevated LVEDPs, and as the latter are being transmitted backward, pulmonary congestion/edema ensues [67, 305]. Notably, as a matter of fact, venoconstriction is part of the “generalized “vasoconstrictive transaction [60, 306, 307].
Cotter demonstrated that almost always systemic vascular resistance is enhanced in acute heart failure syndromes (AHFS) [13, 137] and argues that most episodes of AHF (about 70%) are attributed to increased aortic input impedance [308], which denotes enhanced afterload, with consecutively increased end-systolic LV-stiffness and reduced diastolic compliance [151], resulting in a noticeable rise in LVEDP [113, 127, 145, 146]. This opinion is agreed by Metra and co-workers [160] and supported by several facts, particularly:
(1) Relatively high systolic BPs are frequently seen in AHF patients [15, 69], at least 50% of all patients admitted with AHF are “hypertensive”—systolic BP > 140 mmHg [69, 309]. However, patients with systolic BPs of 140 mmHg or less may very well suffer from augmented afterload as in the setting of systemic inflammation/infection with increased central aortic stiffness and thus increased systolic LV-load [13, 282].
(2) Furthermore, AHF often develops rapidly, typically within hours [17] and without much previous complaints [4, 137], and as such is associated with acute alterations in arterial loading conditions directly affecting cardiac properties and function [160, 308]: “ LV performance is influenced by arterial load [284] (since systolic wall stress reflects afterload as defined by the law of LaPlace [310, 311]), and arterial properties are, in turn, influenced by LV performance” [284, 312]. Consequently, vascular properties (specifically vascular tone) play an essential role in the development and progression of HF [174]. Moreover, (worsening) vascular failure is considered to be a precipitant for AHF [313].
As evident from the above depicted setting, altered vascular properties are decisively causally responsible for incipient AHF, accordingly, this type of AHF is referred to as “vascular failure” or “vascular type of AHF” in contrast to “cardiac failure” [308]. The latter, in which predominantly the cardiac performance and/or the myocardial efficiency is/are considerably compromised, may further deteriorate (e.g. due to ischemia with hibernating or stunning myocardium and thus (further) afflicted contractile properties [314], a common reason in de novo AHFS [26, 33]), and consecutively provoke AHF [308]. Of prominent concern are elevated troponin levels indicating myocardial damage, found in about 50% of all patients admitted with AHFS [315]. These elevations are suggested to be largely attributed to improper and disproportionate myocardial perfusion provoking ischemia (subendocardial ischemia due to high filling pressures, dysregulated cardiac/myocardial autoregulation (altered microcirculation), metabolic imbalances, hypotension, application of vasodilatory substances treating AHF, etc.) [26, 66, 68, 316–318], and will, in any case, (further) weaken myocardial performance [319]. Characteristic for the cardiac pathway (cardiac failure) is a marked de novo fluid accumulation, developed often over weeks, and relevantly involved cardio-renal features [17, 54, 164, 308, 320].
The concept by Cotter takes into account that (A) the heart and the vessel system have to be acknowledged as a functional unit, an absolutely essential view for understanding and interpreting the basic physiological and pathophysiological features, affecting each other and are together responsible for sufficient cardio-vascular performance [147, 312, 321–325], and that (B) both, an afflicted heart and altered vascular properties are present in acute heart failure. However, while one part of the unit may predominantly malfunction in the acute condition, the unit as a whole precipitates the failure [326]. Metra emphasizes, it is even “essential for understanding and treatment of heart failure” to distinguish between both pathways, although they may be disrupted concomitantly and thus contribute equally at the same time to acute decompensation [160]. Further details of this new fundamental concept on AHFS by Cotter, meanwhile recognized and even endorsed by the ESC [5] are depicted in Fig. 2.1.
The vascular pathway is related to increased vascular stiffness/resistance with acute afterload mismatch and exacerbated filling pressures in the setting of activated NHs, resulting in (further) impaired systolic performance and redistribution of fluids from systemic (predominantly splanchnic veins, see below) to pulmonary circulation, rather than from general fluid accumulation. The cardiac pathway implies largely myocardial issues (due to acute ischemia, acute myocardial infarction, or acute myocarditis, but also due to sepsis and other threats affecting myocardium) in the setting of stimulated NHs (further) blunting and deteriorating systolic performance, to primarily responsible for the AHFS and is essentially associated with de novo fluid accumulation and cardiorenal dysfunction [104, 126, 154, 308].
Characteristic for the “vascular profile” of AHF development are rapid onset of clinical symptons and central fluid redistribution causing pulmonary congestion or edema rather than fluid accumulation, thus no or only marginal weight gain prior to AHF is seen, furthermore, most patients have preserved EF, suffer from diastolic dysfunction, and present with normal (sBP 100–140 mmHg) or elevated (sBP > 140 mmHg) blood pressure [17, 308]. Of course, especially affected are in general patients with pre-existing combined v-a stiffening, demonstrating amplified changes for any alteration in loading conditions [147], and ADHF arises due to temporary exacerbated/worsened diastolic dysfunction [127]. As such, acute increases in afterload (e.g. due to an increase in vascular resistance and / or in vascular stiffness) have a markedly unique impact on blood pressure and consecutively on filling pressures [145, 146] since blood pressure feeds back into (further) impairment of diastolic properties [151], potentially inducing pulmonary congestion [112, 327, 328]. Petrie established an inverse relationship between diastolic relaxation and afterload in hypertensive and non-hypertensive humans indicating cross-talk between arterial afterload and diastolic LV function [329]. Clinical pulmonary congestion (compared to hemodynamic congestion which is reflected by elevated LVEDPs but without clinical symptoms [153]) or even edema may apply in case of acutely increased LVEDPs already at relative low filling pressures, and consecutively, relatively lower pulmonary pressures than found in chronically elevated LVEDPs, as pulmonary lymphatics drain excess lung fluids in case of chronic overfilling more rapidly and efficient than in case of abruptly enhanced fluid onset [192, 330].
However, in conclusion, the classical vascular pathway relates to acutely altered vascular arterial impedance (due to increased vascular stiffness and/or resistance) associated with an acute afterload mismatch as the change in loading conditions cannot be compensated by adjusting cardiac performance, the latter in general due to compromised systolic cardiac performance or limited systolic reserve, further affecting systolic properties, and is accompanied by fluid redistribution (due to neurohormonal drive, read below) rather than by fluid retention [117, 154, 308, 331].
2.4.2.4 Fluid Redistribution, Splanchnic Veins and the Venocentric Input
Highly remarkable, very recent study results provide evidence that even subtly altered hemodynamic conditions may provoke AHF from vascular type in predisposed subjects with abnormal cardio-vascular function, whereupon acute sympathetic discharge mediates acute changes in vascular properties with subsequently modified loading conditions thereby leading to acute heart failure [60, 123, 126, 332]. The neurohormonal systems are, as described above, decisively controlling and modulating cardio-circulatory function [183, 184], and may acutely affect cardiovascular conditions and function [164, 186]. Particularly the sympathetic nervous system (SNS) is reported to be able to instantaneously affect cardiopulmonary and arterial baroreflexes [333, 334], and to acutely release vasoactive agents such as noradrenaline into the circulation and thus mediate and induce rapidly changes in vascular arterial and venous resistance and compliance [60, 335]. As such, abruptly enhanced sympathetic drive is demonstrated to critically influence the pathogenesis and onset of AHFS [60, 126, 165, 224, 226]: Study results and considerations by Fallick and colleagues address sympathetically-mediated central fluid redistributions from the splanchnic venous blood reservoir, caused by acutely elevated sympathetic discharge resulting in ADHF [60]. More than 70% of total body blood volume is mainly residing in the veins and not involved in effective circulation, since the venous system is substantially more compliant (about 30 times) than the arteries [335]. Of which, the splanchnic veins are even more compliant than the other veins and are furthermore particularly densely equipped with α1 and α2 receptors [335]. These anatomic facilities translate into physiological consequences, displaying better storage abilities (reservoir veins) than other veins and a significant stronger degree of vasomotor response in case of sympathetic activation [336], which predominantly reduces venous compliance and as such diminishes the vessels’ capacitance [60]. Hence, even minor sympathetic discharges prevailing, translate into constriction of the splanchnic veins while there is no, or only a negligible, effect to be seen in the other veins and hardly any in the arteries. Furthermore, the amount of fluids shifted in case of constriction of the splanchnic veins is comparatively greater as with an equal strong constriction in other venous areas.
Moreover, while the capacitance of the peripheral veins is reported to be normal in heart failure patients [337], it is suggested that splanchnic veins behave dysfunctional in that patient group unable to properly buffer changes in effective circulating volume [60]: The inhibitory control mechanisms, in particular reflex control, attenuate and modulate SNS discharge and cause its effects to not properly apply [338–341]. Adamson provided evidence that imbalanced autonomic activities prefer sympathetic over parasympathetic activity [342], and as such result in sustained sympathetic influence and activism.
Accordingly, sympathetically-mediated and initiated reduction in splanchnic venous capacitance may provoke relevant fluid shifts from the venous reservoir into the effective circulating blood stream, subsequently increasing preload and consecutively enhancing LVEDPs: Indeed, pulmonary diastolic pressures are demonstrated to fluctuate markedly during the day, apparently attributed to sympathetic discharges in response to in principal physiologic matters like upright posture and exercise [332]. While many of these sympathetic discharges are uncritical, some may initiate a vicious cycle ending up in acute heart failure in susceptible persons [60]. Patients suffering from chronic heart failure show chronic endothelial dysfunction and low grade inflammation [80, 175, 261, 343, 344] and as such are predisposed to decompensate—the vast majority (about 75%) of patients with AHFS are acute decompensations of chronic heart failure [345, 346]—in case of a further threat/threats (e.g. temporary ischemia—cardiac pathway) or even minor alterations in loading conditions (vascular pathway) [297]. Accordingly, Fallick and co-authors suppose that acute (and maybe even “physiological“) sympathetic discharge, at least in the setting of dysfunctional splanchnic veins as found in (chronic) cardiac/cardiovascular dysfunction, alone has the potential to provoke AHF [60]. The concept is well consistent with other study results: Autonomic imbalance and elevated filling pressures become evident already days and weeks before acute decompensations turn into a clinically overt malady (display clinical congestion) [123, 342]. Furthermore, elevated pulmonary pressures are suspected to promote sympathetic excitation through pulmonary afferents [347], thereby amplifying sympathetic drive and thus may intensify venoconstriction and consecutively a fluid shift. The results from the COMPASS-HF study suggest, providing clinical evidence, that fluid shifts from the extracellular space into the effective circulation (expanding effective circulating volume) may underlie the development of AHF [348]. Moreover, the authors’ concept also explains very well why patients without weight gain (weight gain is acknowledged to indicate fluid accumulation in heart failure patients, although this is a relatively insensitive (and nonspecific) marker of fluid agglomeration with several limitation and restricted accuracy [160, 349–352]) may well develop AHFS without typical precipitants due to minor, elusory or even not comprehensible occasions initiating incipient acute heart [60, 126]. This pathogenesis explicates that the majority of patients presenting with AHF do not suffer from clinically comprehensible fluid accumulation and weight gain (inducing acute decompensations and AHF [123, 134, 353]), rather, fluid redistribution from peripheral, namely splanchnic venous, to central circulation is definitely an established pathway in AHF pathobiology [60, 126, 160, 308].
Furthermore, this approach broadens Cotter’s concept who attributed fluid redistribution to increased vascular resistance and stiffness, thereby referring vascular failure to altered arterial properties. Obviously, changes in venous tone and hence venous capacitance (namely in the compliance of the splanchnic veins reducing their capacitance due to acutely increased sympathetic drive) foremost apply, and are able to shift within seconds up to 800 mL of blood into the circulation [335], thereby augmenting effective circulating blood volume and concomitantly increasing preload, and thus cause acute heart failure [60, 126]. In summary, sympathetically–mediated veno-constriction, predominantly affecting the splanchnic veins, with subsequent considerable blood shift into the effective circulation and hence increased preload causing (further) elevations in filling and concomitantly pulmonary pressures, inducing pulmonary venous congestion, has to be considered primarily as a vascular pathway with fluid redistribution following Cotter’s concept.
2.4.2.5 Fluid Accumulation, Venous Congestion and the Link Between Cardiac and Vascular Pathway
Expansions, even very mild ones, of the effective circulating blood volume, inevitably increasing the preload, are in the setting of heart failure in any case accompanied by appreciable increases in filling pressures, actually even exponentially increases may be seen [354]. This condition is referred to as hemodynamic congestion [153]. As such, acute increases in venous return, due to reduced venous capacitance of the venous reservoir following sympathetic activation, are able to provoke (occasionally substantial) enhancements in LVEDP and RVEDP [60]. If the increase in cardiac filling and intravenous pressures (elevated left-sided pressures are usually responsible for increased systemic venous pressures [124, 355, 356]) become clinically obvious by precipitating acute pulmonary congestion/edema [60, 226] and systemic peripheral edema (the latter traditionally known and referred to as venous congestion [134, 139]), clinical congestion applies [153]. “Central (pulmonary and intrathoracic) and peripheral (venous) congestion usually exist together” [126, 155, 156]: Pulmonary and systemic congestion caused by elevated left- and right-heart filling pressures is almost a universal finding in AHFS [15].
Congestion is almost always associated with excess extracellular fluid and blood volume [60], whereupon most of the excess fluid will be located in the venous system [335]. However, increased intravascular fluid volume does not always reflect fluid accumulation or retention rather may be due to altered fluid distribution, redistribution, as described by Fallick and coworkers [60] and as conceptualized for AHFS by Cotter [308]. Indeed, elevations in filling pressures following fluid shifts from the venous reservoir may result in a failing heart in hemodynamic or even clinical congestion without any relevant (at least for us recognizable) supplementary retention of salt and water [357]. Consistent, weeks before overt AHF ensues, autonomic imbalance and elevated filling pressures are demonstrated [123, 342]. As such, inappropriate autonomic regulation of the vascular, namely of the venous tone, and the physiological fluctuations in SNS drive, may induce (repetitively) some degree of fluid shift especially from the splanchnic reservoir to the effective circulation thereby potentially provoking incipient AHF [297, 357].
Accordingly, although in the majority of AHFSs (acute) central fluid redistribution, rather than fluid accumulation, is the recognized flash point leading to acute clinically relevant and overt malady [126, 160, 308], at least some degree of fluid excess, often beyond clinical comprehensibleness, is universally present in all AHF patients [35, 126, 358, 359] and a “basic and fundamental mechanism of decompensation” [160]: (1) Sympathetic excitation is reported to facilitate sodium retention and as such may contribute to decompensation [60]—enhanced sympathetic drive is an acknowledged issue in heart failure [227]. (2) Diminished natriuresis is a hallmark of heart failure and thus fluid retention has to be anticipated in heart failure patients [104, 105]. Threats, often minor ones, or even intense physiological fluctuations in the concentrations of (circulating) neurohormones are demonstrated to promote fluid retention in patients suffering from chronic heart failure [55, 59], and may launch acute decompensations—remember, the vast majority (75% and more) are acute decompensations of chronic heart failure cases [346]. (3) Arginine- vasopressin- mediated reabsorption of free water is reported to be present in heart failure [224, 349]. (4) Not only Silva Androne could verify that intravascular volume indeed is elevated in patients with “stabile” chronic heart failure [360]. (5) Furthermore, renal dysfunction is common in heart failure resulting in salt and water retention [224, 349] and may contribute to fluid overload. Actually, in a large majority of heart failure patients, a shortened kidney function has to be recognized [35, 353, 361]. The complex pathophysiology of kidney dysfunction associated with heart failure, the cardio-renal-syndrome (CRS), is largely attributed to a decrease in renal perfusion pressure, altered intrarenal hemodynamics, and elevated renal venous pressures [362], basically a result of the effect of activated neurohormonal systems (SNS and AII !) and associated fluid retention [199, 363], and deficient/overwhelmed counter-regulatory systems and effects [186, 224]. Furthermore the signalling pathways of the natriuretic hormones must be affected in heart failure, since in case of increased natriuretic levels, physiologically an accelerated natriuresis applies [364]. Thus, in heart failure, salt excretion in general is disturbed [126]. (6) Moreover, in the setting of an increased vascular tone which is accompanied by a diminished (foremost splanchnic) venous capacitance [60, 335], the hemodynamic effect of sodium and (consecutively) water retention may be amplified [365, 366]. (7) Especially to be noted, “fluid redistribution can only happen on the basis of an existing elevated blood volume” [126]. Thus some degree of fluid accumulation is necessary for the concepts of Fallick and Cotter to work, explaining very well the pathophysiology in absolute consistency with the clinical findings and presentations.
Nonetheless, fluid accumulation as the typical, classical feature characterizing the cardiac pathway of AHFS following the concept by Cotter [308], applies predominantly in case of relevantly compromised cardiac function [308]. In the setting of markedly impaired, prevailing cardiac performance, progressive fluid accumulation occurs as the result of cardiac failure. The ensuing, often persisting and thereby maladaptive, compensatory mechanisms, including activated neurohormonal cascades and endothelial—inflammatory programs, affect salt and water balance and renal function, clinically manifesting in a more gradual increase in total body volume, with concomitant enhanced body weight and in the front peripheral edema, jugular venous congestion, hepatomegaly, and gut discomfort [5, 17, 50, 308]. As the renal dysfunction and the modified fluid—salt balance component are relevantly involved [124], some authors talk about a cardio-renal pathway (instead of cardiac pathway) [54, 164, 297]. This “slow” decompensation over days and often weeks [134, 342] has been traditionally attributed to non-adherence in diet (improper high salt and fluid intake) and medication, as well decreasing contractility due to ongoing myocardial injury (mainly ischemia) [17, 55, 308]. This pathway is related to largely altered systolic, myocardial properties while changes in, and the impact of, the vascular conditions are seen in these circumstances in the background [308, 311].
Increased intravascular, thus particularly intravenous fluid content exerts biomechanical stress on the vessel walls [259, 367, 368]: Biomechanical forces including shear and circumferential wall stress as well as increased intravascular fluid content precipitating hydrostatic pressure display endothelial stretch, sensed by the mechanoreceptors located on the surface of the endothelial cells [296, 369–371]. Consecutively, the endothelial cells will be activated and hence switch phenotypically, altering their synthetic profile, and as such, physiologically deploy a signalling cascade resulting in a minor degree of vasoconstrictive, pro-coagulatory and pro-inflammatory condition [296, 369–371]. The reaction may be somewhat more pronounced in case of already enhanced vascular tone as typically present in “compensated” chronic heart failure [183, 184, 227]. Moreover, excessive and sustained activation is in any case crucial for disease progression [183]. As such, environmental cues may cause apparent or subtle, unrecognizable biomechanical stress which is associated with endothelial and neurohormonal activation and concomitant generation of oxidative stress. Oxidative degradation of the ROS’s quenches NO (the key molecule of vasodilatation) activity despite elevated NO production and thus results in blunted NO bioavailability. This then affects vascular tone, causing (and amplifying) vasoconstriction [201, 261]. The vasoconstriction may be more distinct and amplified by other neurohormones modulated and released following vascular stretch, notably the renin-angiotensin-aldosterone—system with angiotensin II [259, 297] as its biologically most active representative, which, in turn, directly and indirectly promotes vasoconstriction [372]. Especially to be recognized, peripheral rather than central, cardio-pulmonary triggers are reported to be the decisive source of activating endothelial cells to generate and release vaso- and bioactive mediators [307, 373]. However, that is not surprising and absolutely consistent with the natural physiological conditions as most of the accumulated or retained excess fluids are “stored” within the venous system [335]. Accordingly, systemic, particularly local venous hemodynamic and finally clinical congestion (venous congestion, mainly a result of the activated compensatory mechanisms and cascades, is associated with circumferential stretch [259]) causally accompanies and potentially facilitates acute heart failure evolution [54, 164, 174, 186, 192], as congestion is considered to play a crucial role in provoking endothelial and neurohormonal activation [374, 375]: “Systemic venous congestion is sufficient to cause endothelial and neurohormonal activation” [297]. However, as Hayashi [307] could demonstrate in a human study model of patients with systolic heart failure (HFrEF), local venous congestion is also, by all means, able to “promote endothelial and neurohormonal activation, even exerting systemic effects, as evidenced by an increase in plasma ET-1, IL-6, and VCAM-1 in this patient population” [297]. The special input from venous congestion in the acute and chronic heart failure pathobiology is further supported by the fact that venous congestion commences, and can be observed, days and even weeks before clinically overt heart failure ensues [133]. Hence, venous congestion has to be considered as being itself a primary contributor and hemodynamic, pro-oxidative, and pro-inflammatory stimulator of acute decompensation, rather than an epiphenomenon and merely a consequence of poor cardiac performance [133, 297]. Accordingly, there is substantial evidence that venous endothelial stretch, associated with and caused by local (and systemic) venous congestion following fluid retention and accumulation [259, 297, 307], is able to activate particularly the local, peripheral venous endothelial cells to subsequently produce and release, in a paracrine/endocrine manner, a number of vaso- and bioactive mediators and substances including vasoactive and inflammatory neurohormones and cytokines [259, 297, 374, 375] in a composition compatible with results typically demonstrated in AHFSs [374, 376]: “The peripheral release of vasoactive and pro-inflammatory neurohormones and substances from stretched endothelial cells and perivascular congested tissues may offset the physiologic adaptive state and may promote further fluid retention (fluid accumulation) inducing a vicious cycle resulting in overt decompensation” [375].
Thus, in consequence of the extended and elevated intravascular, namely intravenous, fluid amount, a rise in filling pressures of both the right and the left ventricle ensues. Subsequently progressive central pulmonary and peripheral local and systemic venous congestion will be displayed [104, 117, 126, 377]. Pulmonary (left-sided) and systemic venous (right-sided) congestion are related to elevated left- respectively right- sided filling pressures [15], whereupon the elevated LVEDP is the characteristic consequence of the systolic and/or diastolic cardiac dysfunction causally present in heart failure syndromes [378]. Elevated right-sided filling pressures are the result of (a) the elevated LVEDP being transmitted backward to the pulmonary vessel network, causing pulmonary venous hypertension, consecutively increasing RV-afterload [379, 380], (b) the increased RV preload and (c) of diastolic ventricular interaction applying in the presence of (acute) heart failure and typically if intravascular fluids distinctly accumulate as in cardiac malfunction [131, 132]: In circumstances with relatively preserved RV function, the failing left ventricle, unable to properly accommodate with any accessory fluid without a rise in LVEDP [354], responds with a further (often inappropriate high) increase in LVEDP, even if the fluid amount offered by the RV is small [117, 125, 354] affecting markedly RV afterload and filling characteristics [380]. Accordingly, besides the enhanced right ventricular filling “inherently” promoting an increase in RVEDP [381, 382], and the backward transmission of elevated LVEDP first and foremost contributing to an enhancement of RVEDP [67, 383, 384], DVI will contribute to a recognizable, sometimes marked increase in RVEDP [132, 385, 386]. In case RV function is also altered, the increase in REVDP will be accentuated [385–387] and is typically higher than the rise in LVEDP [385, 386], as notably diastolic ventricular interdependence will decisively influence the filling pressures [131, 388, 389]. Accordingly, in most cases (≫80%), the increase in LVEDP is accompanied by a noticeably substantially elevated RV-filling pressure [121]. Indeed, Gheorghiade found that nearly all patients suffering from acute heart failure present with both, systemic and pulmonary congestion [153]. Anyhow, subsequently RV function [390, 391] as well as diastolic [392] and systolic [393–395]) LV properties will be further compromised.
Hence, a considerable increase in LVEDP following enhanced intravascular volume arises, potentially causing clinical pulmonary congestion or even provoking pulmonary edema [125, 396–399]. Pulmonary congestion is associated with reduced oxygen saturation and myocardial ischemia potentially arises if oxygen saturation is less than 90%, furthermore circulatory insufficiency results in metabolic acidosis which jeopardizes the heart [137].
In addition, venous congestion (which is affected by the amount of intravenous fluid volume, changes in venous tone and sympathetic activation [60, 400]) is demonstrated to impair cardiac function [125, 126, 401] (another hint that venous congestion is a contributor rather than simply an epiphenomenon of AHF), and increases in LV end-diastolic filling themselves inherently augment ventricular stiffness (and thus afterload) and decrease EF [126].
Hemodynamic congestion may be seen as fluid retention and occurs early in the course but is clinically imminent [153]. However, local peripheral venous distension and local tissue edema, both attributed to enhanced fluid content, result in venoconstriction, since endothelial stretch initiates local (but accompanied by systemic) neurohormonal and endothelial activation, where particularly A II, ET-1 and sympathetic discharge are responsible for the local venous venoconstriction [174, 261, 297, 402, 403]. This will precipitate further fluid influx, preferentially from the splanchnic veins, enhancing effective circulating blood volume [60] and promoting a further increase in venous return and thus preload, but as well amplifying venous congestion. As a matter of fact, there will arise a somewhat worsened/affected arterial stiffness due to the inflammatory effects displayed [282]. Both effects, venoconstriction with associated fluid shift and the (more) stiffened arteries (further) alter loading conditions and effect systolic and diastolic ventricular properties translating in a further increase in LVEDP (RVEDP respectively) and compromised LV and RV function [15, 60, 134, 153, 284, 285, 297, 354].
Very remarkable, these considerations are not only indicative for a vicious cycle being established and applied leading to acute decompensations and disease progression, but are rather well suggestive of a link between fluid accumulation and vasoconstriction, notably venoconstriction, the latter affiliated with fluid redistribution. Hence a link between the vascular and the cardio-renal pathway [297]: Venoconstriction due to neurohormonal, namely sympathetic discharge by reducing the venous capacitance [60, 335, 404], induces a fluid shift, preferentially from the splanchnic venous reservoir, into the effective blood circulation, hence central fluid redistribution applies. Subsequently a rise in preload and inevitably an, often marked, increase in filling pressures ensues, facilitating further venous congestion through fluid accumulation [60, 153, 297, 374]. On the other hand, fluid accumulation, primarily due to impaired (and during the course progressively worsening) cardiac performance, leads to (further) endothelial and neurohormonal activation, ending up in a pro-inflammatory, pro-coagulative and vasoconstrictive milieu, fostering vaso-, especially splanchnic venous, constriction. The splanchnic veins are shown to be exceptionally sensitive to even discrete sympathetic discharge, and as such shift and redistribute blood from the venous reservoir into the effective circulating stream. Augmented preload and concomitantly filling pressures result [60, 133, 297] (Fig. 2.2).


Fig. 2.2
Adapted from Colombo PC (Curr Heart Fail Rep 2015; 12: 215–222) [297], with permission. Depicted is the suggested link between fluid redistribution and fluid accumulation, both effective in acute heart failure pathobiology, independent of the threat primarily launching the decompensation. The pathways are linked and affect each other. The finding that venous constriction may also precipitate central fluid redistribution broadens Cotter’s concept [308], and explains well that even minor (repetitive) sympathetic discharges may lead to acute decompensations—and that without noticeable fluid accumulation
With these remarks it becomes obvious, that even mild, primarily natural and reasonable modifications, adaptations (e.g. to upright position) within the physiological range may offset (“just”) compensated conditions initiating a vicious cycle in which (further) sympathetic discharge and other regulatory cascades lead to and provoke acute heart failure [126, 297]. Furthermore, Fallick’s [60] and Colombo’s [297] considerations add a new aspect to the existing views and concepts, namely a special venocentric approach (in addition to cardiocentric, nephrocentric and arteriocentric): Venous congestion contributes (via triggering local and systemic endothelial-inflammatory response and compensatory mechanisms) to the heart failure pathobiology by promoting (further) increases in effective circulation blood volume with consecutively increased preload, filling pressures, aggravated congestion and additional fluid accumulation.
2.4.2.6 (Self)-Amplification and Vicious Cycles
Furthermore, as exemplarily demonstrated by the link between the vascular (vasoconstriction and fluid redistribution) and cardiac/cardiorenal (fluid accumulation and venous congestion) pathway, and as demonstrated by the interconnections of the neurohormonal and endothelial-inflammatory features and systems involved, this condition bears a considerable potential of self-amplification and perpetuation of a vicious cycle driving the heart failure malady [186, 297]. As such, elevated, high LVEDPs and myocardial stretch, typically present in acute (and chronic) heart failure, are known to be very powerful biomechanical incentives. They cause neurohormonal activation including the adrenergic and cytokine pathways and the RAAS, [104, 160, 405], provoke subendocardial ischemia (further affecting the cardiac properties) [66, 68] and reduce coronary perfusion (potentially causing ischemia) [160], not at least facilitate changes in LV-shape and thus functional mitral regurgitation (affecting hemodynamics) [36, 406, 407]. Furthermore, simply ordinary stress may induce an increase in LAP/LVEDP causing further distress in predisposed patients with neurohormonal activation, and in consequence facilitate congestion [297]. Moreover, in the setting of an increased vascular tone which is accompanied by a diminished venous, foremost splanchnic, capacitance [60, 335, 404], the hemodynamic effect of sodium and (consecutively) water retention may be amplified [365, 366].
2.4.3 Summary
“The syndrome of heart failure is the result of complex interactions among molecular, endocrine, and biodynamic systems” [408]. The intricate pathophysiology is of multifactorial and multi-facet nature, however appears to be largely related to the complex interplay between neurohormonal activation and adaptive remodelling efforts with the mechanical-hemodynamic disorders [288, 409]. Typically characteristic for ADHF is “a mismatch between loading (pre- and/or afterload) conditions and the afflicted, impaired cardiac function” [160, 297, 308]. Indeed, the communication, the cross-talk, between vascular and cardiac properties considerably determines the circulatory conditions [53, 54, 410, 411] of this systemic disease [412–414], which may affect over time several organs, preferentially the kidneys [98, 400, 415].
Two mainstream pathophysiological pathways applying and leading to AHF were recently introduced by Cotter allowing for integration and harmonization of the clinical pictures with the pathophysiological concepts [308]: Vascular failure precipitating and ending up in AHF describes alterations in vascular properties modifying systolic and/or diastolic loading conditions, evoking an acute mismatch as the systolic and/or diastolic cardiac capacities and capabilities are primarily impaired or limited and thus not able to properly meet the altered conditions [60, 126, 153, 297, 308, 357]. This path is associated with central, pulmonary fluid redistribution rather than with fluid accumulation and has been originally related to predominantly (acutely) increased arterial vascular stiffness/resistance causing an acute afterload mismatch [117, 160, 308]. As such, it applies particularly to patients with diastolic dysfunction and HFpEF [112, 113, 127, 148, 214, 322]. However, this vascular failure path has been broadened and modified as even subtle hemodynamic changes, often affecting primarily preload conditions, may already precipitate AHF [126]: Sympathetically-mediated vasoconstrictions, preferably due to the anatomic circumstances, may be exclusive to the splanchnic veins (and thus a vascular path), and are shown to shift relevant amounts of the their stored blood into the effective circulation, subsequently increasing cardiac preload [60]. As the compromised left (and often right) ventricle cannot accommodate any increase in filling volume without often marked rises in filling pressures [354], consecutively clinical pulmonary and venous congestion arise and overt acute heart failure ensues [67, 104, 117, 126, 135, 153]. In predisposed patients (patients with chronic heart failure and chronically augmented NHs drive) minor sympathetic discharges, even physiological ones due to mild exercise or posture, upright positioning [332] may, particularly if repetitive with repeated blood volume shifts into the effective circulation [123], (ultimately) provoke AHF—this scenario, which may also apply in case of decompensations without evidence for classical precipitants, explains well that the majority of patients with AHF do not show any or only marginal weight gain prior to decompensation [60, 126, 357].
The cardiac or cardiorenal pathway represents the classical pathomechanism of AHF [308]. Related to considerably impaired cardiac performance (and thus systolic dysfunction, HFrEF), the hemodynamic alterations and associated, the activated, basically compensatory systems, lead to substantial fluid accumulation [5, 17, 50, 308], often developing slowly and gradually [123, 342]. Fluid accumulation, is, at least to some degree (including in the predominantly vascular failure path), in general part of the heart failure pathology [35, 126, 358, 359], although definitely quite often not obvious for us, as it is not measurable by methods applicable in daily practice (as it may be without relevant weight gain) [160, 349–352]. However, increasing intravascular fluid volume precipitates an increase in preload and venous congestion, the latter meanwhile verified to be an active contributor to heart failure pathobiology [133, 297], and furthermore challenges even more the neurohormonal (namely sympathetic and A II discharge) and the endothelial-inflammatory paths [297]. Again, the increase in filling volume, preload, and consecutively pulmonary and systemic pressures lead to overt clinical heart failure [104, 117, 124, 126, 135, 153, 355, 356]—an increase in LVEDP may be due to fluid redistribution and/or due to fluid accumulation [117]. Furthermore, venous congestion is demonstrated to (further) impair cardiac function [125, 126, 404] and to foster (further) fluid accumulation [60, 153, 298, 377].
Accordingly, both pathways are linked and interrelated [297], moreover their interaction “may promote a vicious cycle as fluid accumulation causes vasoconstriction”, more precisely predominantly venoconstriction, “while vasoconstriction causes an increase in filling pressures” (via increased preload due to blood shift from the splanchnic veins) “and thus promotes (further) venous congestion through (further) fluid accumulation” [297].
The described modifications, adaptions, reactions, and altered conditions are partly due to, but in any case largely mediated, coordinated and integrated by the activated NHs and endothelial—inflammatory paths [165–169, 173, 174, 416], both with substantial impact on the cardio-circulatory system [183, 186], and with the endothelium playing a central role in the pathobiology of acute and chronic heart failure, “orchestrating” the processes [173, 175, 223, 416] .
The central feature related to the underlying primary pathologies and the incorporated multifactorial patho-biological processes, markedly elevated left- and generally right-sided filling pressures, which are affiliated and associated with pulmonary and systemic venous congestion (independent whether CO is low or not) [14, 15, 122–124, 153], are displayed [14, 122, 153, 154, 214, 378]. These elevated pressures may be the pivotal position in the pathophysiology and are decisively determining and coining the clinical picture [5, 17, 50, 106].


Fig. 2.3
Fig. 2.3 summarizes the central pathobiology of acute heart failure syndromes. It is mainly based on the publications by Cotter [308], Paulus [37], Fallick [60], Metra [147], Borlaug [147], and Colombo [297]. Modifying Cotter`s fundamental concept, it integrates the most recent pathophysiological findings contributing to and co-determing acute heart failure. It depicts that indeed one of the basic malfunctions/insults (altered loading conditions or impaired systolic performance) predominantly activates and launches certain pathobiological processes, but highlights the close interactions between the two paths and their potential to amplify each other, and thus facilitate the evolution of AHF
2.5 Diagnosis, Symptoms, Presentation, Important Clinical and Prognostic Data
2.5.1 Symptoms and Diagnosis
The diagnosis of acute heart failure is generally based on clinical symptoms (dyspnoea, orthopnoea, shortness of breath on) and signs (crackles on pulmonary auscultation, peripheral edema) suggestive for heart failure [14, 417] in the context of clinical history, physical examination and other findings [6, 418, 419]. Dyspnoea is the most common symptom, however it is non-specific. On presentation [14, 28, 44, 49]:
up to 89% | Suffer from | Any dyspnoea |
up to 34% | Dyspnoea at rest | |
up to 32% | Fatigue | |
up to 68% | Rales on examination | |
up to 66% | Peripheral oedema | |
up to 75% (60–90% [49]) | X-ray congestion |
Symptoms and signs like paroxysmal nocturnal dyspnoea attacks, jugular venous distension, and third heart sound S 3 are quite frequently seen as having a specificity of 70–90%, but a really low sensitivity 11–55% [420].
Thus, symptoms are dominated by those related to pulmonary congestion, reflecting the elevated LVEDP [14, 69].
Insofar the pulmonary affliction attributed to the left heart disease may contribute to the patient’s symptoms and the clinical picture: The pulmonary mechanics are affected leading to a reduction in lung volume and a diminished lung compliance, thus displaying a restrictive lung physiology [421, 422]. Furthermore, gas exchange is hindered [421, 422]. While fluid removal improves lung mechanics, a dysfunction of the alveolar membrane diffusion capacity will persist in the first instance [423, 424].
Blood pressure on admission both provides information on prognosis, and smooths the way of therapeutic measures [5, 35, 425].
Blood pressure ranges on admission are distributed as follows [14, 28, 33, 44, 69]:
sBP > 140 mmHg | 50% of all admissions (approximately 25% have a sBP > 160 mmHg) |
sBP 90–140 mmHg | 45% of all admissions |
sBP < 90 mmHg | 5% of all admissionsa |
However, high BP on admission may be due to sympathetic stimulation rather than established hypertension [69].
Dominant clinical conditions on admission to hospital in the Euro Heart Survey [28] (see ESC classification [4]):
66% presented with the picture of acute decompensated/exacerbated chronic HF;
17% showed pulmonary oedema as the dominating clinical condition;
10% were admitted due to HF and arterial hypertension;
4% with cardiogenic shock;
3% were admitted due to an acute right heart problem.
As mentioned, the French survey [49] published in 2006 included the very sickest patients as well and recognised pulmonary oedema in 82% and cardiogenic shock in 29%.
2.5.2 Prognostic Indicators
The main predictors of prognosis signalizing high mortality are low systolic blood pressure (sBP) and elevated BUN at admission [35, 49, 425].
sBP at admission (mmHg) | In-hospital mortality (%) | 60–90 days mortality (%) |
---|---|---|
≤119 | 7.2 | 14.0 |
120–139 | 3.6 | 8.4 |
140–160 | 2.5 | 6.0 |
≥161 | 1.7 | 5.4 |
Thus, a systolic blood pressure (sBP) ≤ 120–125 mmHg should give cause for concern, and admission to a coronary care unit or high dependency unit should be considered.
2.5.3 Initial Clinical Assessment, Diagnostic Measures and Considerations
The cornerstones in making the diagnosis are the patient’s history and the clinical examination, read above [4, 6, 419, 429–431].
Patients admitted with symptoms generally suggestive for heart failure and a typical history should be subject to a 2-min bedside clinical-hemodynamic examination [158, 431, 432] (see Fig. 2.4). Furthermore, potential conditions triggering acute cardiovascular decompensations have to be identified whenever feasible [431].
2.5.3.1 Hemodynamic Profiles on Admission
A clinical-hemodynamic, widely used in daily practice, and easy to perform assessment tool for patients with acute heart failure syndromes, allowing for a meaningful and crucial distinction of those patients [224], has been introduced by Nohria and Stevenson [158, 426] (see Fig. 2.4). It takes into consideration the most prominent clinical features and basic pathophysiological issues characterizing the nature of AHF, gives a clue about the severity of the actual situation and possible complications the physician may be faced with, like potentially ensuing shock, and provides hints to select therapeutic measures [16, 17, 433, 434].
Accordingly, most patients can be classified during that 2-min bedside assessment [158, 432, 435] into a hemodynamic profile with a corresponding treatment regimen [158]. The main hemodynamic abnormalities are related to filling pressure and peripheral perfusion. In the presence of elevated filling pressures the patient is said to be ‘wet’, in their absence ‘dry’; if the perfusion of the peripheries is adequate, the patient is ‘warm’, if critically reduced ‘cold’. Note that the assessment concerning a ‘cold’ patient due to hypoperfusion should be made by assessing the legs and forearms rather than the feet and the hands [435] (Fig. 2.4).


Fig. 2.4
The 2-min bedside assessment by Nohria and Stevenson [158, 426, 432] allows for a clinical—hemodynamic assessment and classification of AHF patients and furthermore provides therapeutic and prognostic hints. This figure is adopted from the ESC guideline [431]. Depicted are the four different profiles most patients can be assigned to. On the right upper (wet and warm), the patient is predominantly wet and signs and symptoms associated with increased filling pressures and congestion are dominating. Beyond, right lower (cold and wet), hypoperfusion is the dominating clinical impression indicated by the features described
Haemodynamic profiles are:
Profile 1: Warm and dry → will not be seen in emergency admission unit. Requires therapy along standard chronic heart failure guidelines.
Profile 2: Warm and wet (67% of all patients [436]) → main step is the application of diuretics (or the increase the dosage of their diuretic medication), but initially nitroglycerin sublingual may make sense as well as long as sBP is >100–110 mmHg [5, 7, 35].
Profile 3: Wet and cold (28% of all patients [436]) → warm the patient by either using vasodilators (nitroglycerin or nitroprusside) in case of sufficient blood pressure and signs of vasoconstriction, otherwise inotropes and/or vasopressors are required; when this is achieved, dry them with the aid of diuretics.
Profile 4: Cold and dry → they seem often surprisingly stable, but may collapse unexpectedly; therapeutic measures depend on the underlying reasons and conditions and may include diuretics in case of predominant or isolated right heart failure (with DVI) as long as BP is sufficient, or inotropes and/or vasopressors; but sometimes just fluid may be needed.
Clinical symptoms and signs of congestion (wet) include: Pulmonary congestion (crackles and rales), jugular venous distension, peripheral oedema, hepatomegaly, orthopnoea, paroxysmal nocturnal dyspnea, gut congestion and ascites, hepatojugular reflux [4, 30].
Clinical signs of hypoperfusion/shock (cold) comprise the following [158, 437, 438]: Altered level of consciousness (confused, quiet, apathetic, dizzy), cold peripheries (forearms, lower leg), moist and clammy skin, mottled extremities, ↓ toe tip temperature, oliguria (renal dysfunction), narrow pulse pressure, ↓ MAP, hepatic dysfunction, low serum sodium.
2.5.3.2 Identification of Precipitants of AHFS
Typical features precipitating acute decompensations may include:
Ischemia/acute coronary syndromes;
Systemic infections, notably respiratory tractus infections;
Poorly controlled co-morbidities, such as exacerbated COPD with and without pneumonia;
Uncontrolled hypertension/acute hypertensive dysregulations;
Arrhythmias (atrial/ventricular arrhythmias);
Nonadherence to medication;
Renal failure/worsening renal function;
Nonadherence to diet/inappropriate salt intake;
Inappropriate physical stress;
Drugs like NSAID’s, corticosteroids, chemotherapeutics;
Pulmonary embolism;
Enhanced sympathetic discharge as in Takotsubo cardiomyopathy
2.5.3.3 Other Diagnostic Measures
Echocardiography, considered the “gold standard” for the detection of LV dysfunction [442], is an essential tool which should be performed to evaluate LV-function, structure, and any alterations to this; confirmation of the diagnosis (acute) heart failure is essential as well as identifying potentially reversible causes [4, 443]. However, an immediate echocardiographic examination is “only” imperative in patients with hemodynamic instability and in case life threatening conditions are suggested [431]. In de novo AHF, heart ultrasound is recommended to be performed in otherwise stable patients within 48 h [431].
Of note, ultrasound of the lungs to identify congestion and pulmonary edema (and their severity), substantiate [156, 444–446] or even diagnose AHF [447–449] in case the diagnosis is uncertain, may be of great value as recent publications revealed [444, 445].
The chest radiograph will aid diagnosis of congestion and/or pulmonary oedema [42, 82], and may identify cardiomegaly. However, in up to 20% of cases, the chest X-ray in AHF patients may be nearly normal [119].
The electrocardiogram (ECG) will help to identify a precipitating ischemic event or the new onset of atrial fibrillation inducing the AHFS [4, 429]. A normal ECG in a clinically suggested case of acute heart failure virtually rules out this diagnosis [429].
As the symptoms of acute heart failure may be non-specific and as the physical findings are sometimes not particularly sensitive [432, 450], the Natriuretic Peptides, ANP and BNP, may be helpful in the diagnostic and differential diagnostic considerations, particularly in the emergency department [194, 451–453].
It is in particular the excellent negative predictive value of BNP which can be used to exclude heart failure and to differentiate potential cardiac failure from other underlying diseases [454]. On the other hand, elevated levels do not automatically confirm the diagnosis of AHF, as natriuretic peptide serum levels may be enhanced in quite a number of other cardiac (LV hypertrophy, myocarditis, tachyarrhythmias, pulmonary hypertension) and non-cardiac reasons including advanced age, cardiometabolic disorders, severe infections, anemia, renal and liver dysfunction, ischemic stroke and subarachnoid bleed, and in the paraneoplastic syndrome [455–457].
Troponin T and I are highly sensitive and specific parameters, allowing identification of myocardial injury and play a well-established key role in diagnosing acute coronary syndromes (ACS) [458], as well as in the risk stratification and management of patients suffering from ACS [459–462].
An elevation of cardiac troponin is found in about 40% of all patients with acute decompensated heart failure [463, 464], is associated with a low LV-EF [465, 466], and is said to predict a poor short term prognosis [465, 467].
You [468] has shown that troponin I is a strong predictor of all-cause mortality in patients with acute decompensated heart failure. The study shows an independent ‘dose’-response relationship between cardiac troponins and mortality in AHFS-patients. Thus, an association between elevated cardiac troponins and poor outcome in acute heart failure seems to be established [465, 467].
2.5.3.4 Special Remark: Non-invasive Estimation of Cardiac Index
Cardiac output and cardiac index are undoubtedly the parameters widely used in daily practice. In 1989, Stevenson published a method remarkably reliable, able to estimate CI non-invasively: If the ratio [sBP–dBP]/sBP < 25% then CI is highly likely to be less than 2.2 L/min/m2. This prediction shows a sensitivity of 91%, its specificity is 83% [432] (Table 2.3).
Warm and dry | Warm and wet (>50% of all patients) |
---|---|
Clinical: No specific heart failure symptoms; commonly signs of severe infection/sepsis, tachycardia, hyperthyroidism, etc. | Clinical: Symptoms dominated by the ↑ filling pressures causing shortness of breath—pulmonary congestion and/or acute and ‘chronic’ pulmonary oedema, peripheral oedema and ascites; S3 is heard |
Hemodynamics: sBP low n/n/↑ ; CI/CPI n/↑↑↑, PCWP n | Haemodynamics: sBP low n/↑↑↑; CI CPI (↓)/n/↑; PCWP ↑/↑↑↑ |
Hypoperfusion: None Clinical scenarios most likely in this group: none, may be ESC-5, ESC-6, ESC-1? | Hypoperfusion: None to mild; end organ hypoperfusion: (CNS) only in HTNRenal perfusion: discordantly ↓ RBF, impaired intra-renal autoregulation; ↑ renal venous pressure |
Key question: Is the diagnosis AHF correct? | Clinical scenarios most likely in this group: ESC-1, ESC-2, ESC-3; ESC-5 |
Treatment: Treat other predominating non-cardiac disease, fluids | Treatment : Diuretics , and additional vasodilators (GTN) if appropriate (BP still >110 mmHg after diuretics are already applied) like in hypertensive acute heart failure/pulmonary edema |
COLD and dry | Cold and wet (>25% of all patients) |
Clinical: Often stable, symptoms dominated by hypoperfusion such as altered level of consciousness, cold peripheries (forearms, lower leg), ↓ toe tip temperature, oliguria, ↓ MAP, (sometimes unappreciated congestion). May be some occult signs of very mild fluid overload as in patients with significant ↓ contractile capacity and/or severely dilated chambers (ESC-1) otherwise no “specific” heart failure symptoms | Clinical: Dominated by symptoms of hypoperfusion such as altered level of consciousness, cold peripheries (forearms, lower leg) with cold skin, moist and clammy, mottled extremities and ↓ toe tip temperature, oliguria, congestion/pulmonary oedema; ↓ MAP; S3 heard; often caused by AMI |
Haemodynamics: sBP ↓/n; CI/CPI (low n)/↓/↓↓↓; PCWP n/↓ | Haemodynamics: sBP ↓/↓↓↓; CI/CPI ↓/↓↓↓; PCWP ↑/↑↑↑ |
Hypoperfusion: Mild to moderateAltered renal perfusion: ↓/↓↓ RBF, impaired renal autoregulation | Hypoperfusion: Mild to severeRenal perfusion: ↓ RBF; impaired intra-renal autoregulation; ↑ renal venous pressure |
Clinical scenarios most likely in this group: ESC: ESC-6, ESC-1 | Clinical scenarios most likely in this group: ESC-4a and 4b (pre-shock* and manifest CS), ESC-5 |
Treatment: Fluids, inotropic support, vasopressors if sBP < 90 (85) mmHg; but may be diuretics are appropriate as in case “isolated” RV-F or biventricular, predominantly RV-failure with substantial pericardial constraint and DVI | *Pre-shock criteria: Hypoperfusion present but sBP > 90 mmHg, crackles ≥50% of total lung area, pulmonary oedema, cold and sweaty patient, history of previous AMI |
Treatment: coronary intervention in case of AMI; vasopressors (NA) in case of sBP < 90(85) mmHg in order to avoid myocardial ischemia/further myocardial ischemic damage. Inotropes may be considered before NA is started |
2.6 Therapy [4, 5, 7, 431, 432, 437, 469–474]
2.6.1 Therapeutic Principles and Goals
Peripheral, namely pulmonary congestion, or even pulmonary edema, associated with elevated filling pressures decisively coin the clinical picture and dominate the patient’s discomfort and symptoms. Elevated afterload and pulmonary congestion are a key clinical-pathophysiologic features in AHFS [5, 14, 50, 106, 114, 115, 122, 123].
To address the acute malady picture and pathophysiology dominating features, the administration of loop diuretics remain the cornerstone measure [5, 7, 16, 359, 476, 477].
In the ADHERE registry, 88% of all AHFS patients received intravenous (i.v.) diuretics as a first line measure [478]. Since diuretics lead to a very rapid symptom relief and further address the patho-physiological features (fluid overload and elevated filling pressures, both closely related to the clinical pictures), they have gained universal acceptance and priority in AHF treatment. However, no randomized placebo-controlled trials assessing diuretic use in AHFS exist [479]. Moreover, a number of study results even advise against diuretic use, particularly in high dosages, as diuretics may be accompanied by a number of adverse effects and even an increase in mortality cannot be excluded [480–483]. Especially dreaded are induction of vasoconstriction [484] and (relative) hypovolemia due to diuretic application [485, 486], associated with increased mortality rates [482, 487, 488].
Early on, diuretics exhibit vasodilatory effects, thereby causing transient venodilation, immediately lowering right atrial and pulmonary capillary wedge pressure, consequently left-sided filling pressure, thereby mitigating dyspnoea prior to the onset of diuresis [484, 489]. Further on, urinary output increases by excretion of fluid and sodium [433], reducing filling volume and filling pressures, and as such dilute peripheral and pulmonary congestion/edema [490]. Finally, extracellular fluid volume drops and the patients forfeits body weight. Thus diuretics given sufficiently early reduce intravascular volume and filling pressures, as well as peripheral and pulmonary congestion [490].
Even in case of no obvious relevant weight gain prior to decompensation, patients with acute heart failure are basically somewhat volume overloaded, thus diuretic therapy is required and absolutely indicated [35, 126, 358, 359]. Furthermore, in advanced heart failure, with sometimes low normal blood pressures, the application of diuretics has been shown to be pretty safe, as Atherton demonstrated [131]: In advanced heart failure, roughly 50% of the patients suffer from clinically relevant right heart dysfunction/failure. Hence, diastolic ventricular interaction and pericardial constraint apply, affecting the pathobiology [131, 491]. Accordingly, even in case of relatively low pressures (<80–90 mmHg), diuretics are the drugs of choice to improve volume distribution between the ventricles and subsequently hemodynamics, leading to a substantial increase in BP. However, even in case relevant pericardial constraint and DVI are not effective in patients with advanced heart failure, no significant and clinically meaningful side effects have been observed if diuretics were applied [131]. However, keep in mind, patients suffering from HFpEF are exquisitely sensitive to volume and pressure changes and may reply to the effects of diuretic agents with substantial pressure drops [112, 113].
The administration of diuretics is validated and conceded to be a class I, level B evidence of the American ACCF/AHA [7, 319], while the European (ESC) society’s recommendation discloses to apply diuretics as class I, level C [431].
Vasodilators, namely nitroglycerin (GTN), although exerting a direct lowering effect on elevated filling pressures and on the enhanced afterload, provide a ‘physiological’ therapeutic approach [157, 480], but have not gained as universal an implementation and acceptance as the diuretics [5, 7]. Vasodilators promote a rapid normalization of the altered hemodynamics [157, 492], as afterload reduction implies that LVEDP will drop: ↓ afterload → LVEDP ↓ [493]. Furthermore, the failing heart is exquisitely sensitive to afterload [494, 495] and hence a reduction in the LV outflow impedance (afterload) hampering the ejection by pharmacological vasodilatation will improve the LV ejection, and as such will significantly increase the LV-forward output [496, 497]. In addition, they will substantially reduce the regurgitant orifice and the grade of the mitral regurgitation, very often accompanying LV dysfunction [498, 499].
Thus, afterload ↓ → LVEDP ↓ [500, 501] → diastolic wall stress ↓ → O2-requirement ↓ [502] → LVEDD ↓ [500–502], subsequently, afterload ↓ → SV/CO ↑ [439].
Accordingly, the application of vasodilators are a rational and a clinically validated approach to acute left heart failure treatment [5, 7, 431, 503]. However, they do not address fluid accumulation and are associated with an increased risk and incidence of hypotension [84, 504]. Hypotension may jeopardize myocardial perfusion, and by blunting autoregulated cardiac/myocardial blood flow disturbs blood distribution, consecutively myocardial ischemia ensues (or is aggravated), which subsequently dilutes contractility and cardiac performance and that in the presence of an already compromised myocardial function as in heart failure [315, 316, 319, 505]. Furthermore, vasodilators unfortunately could neither provide substantial evidence that they ameliorate symptoms nor that they improve outcome, namely reduce mortality rates [316, 433, 481, 504, 506–508]. Probably therefore, the recommendations to use vasodilators are inconsistent and differ from society to society. As such the American societies ACCF/AHA recommend to use vasodilators “just” as an adjunct to diuretic therapy (class IIb, level A recommendation) [7], while the ESC validates nitrates as a class IIa, evidence level B measure, particularly to be administered in hypertensive patients [5, 431].
Applying the clinical-hemodynamic assessment results using the 2 min bedside tool by Nohria and Stevenson, the treatment of the “warm and wet patient” will need sufficient dosages of diuretics, furthermore hypertensive patients and those with a sBP above 110 mmHg may benefit from additional vasodilators (ESC types 1–3 and 5) [5, 7, 50, 308, 479]. In hypertensive AHF, vasodilators, e.g. GTN, may be initially be preferred [431, 481, 509].
Type ESC-1 may be warm and wet, but could be cold and wet as well. As such, sBP is in general normal, hence those patients will basically be treated solely with diuretics as typically considerably fluid overloaded when acutely decompensated [5, 7, 50, 308, 479].
The “cold and wet patients” are a risky group as they are in, or may develop, cardiogenic pre- and manifest shock. Thus, those patients require thorough monitoring and besides diuretics either vasodilators (if sBP is well above 110 mmHg) or non-vasodilating inotropes (or a combination of noradrenaline and dobutamine) in order to improve perfusion. In case of manifest cardiogenic shock, vasopressor application may be required as the very first measure.
In any case, (further) coronary hypoperfusion needs to be avoided completely, otherwise a progressive detrimental loop resulting in cardio-circulatory collapse and multiorgan dysfunction may ensue. Typically within this class profile ESC-4, ESC-5 (with peripheral edema but clear lungs), but ESC-1 may fit as well [5, 7, 50, 308, 479].
The “cold and dry patient” is rare (typically ESC-6, may be ESC-1, 4 and 5), but difficult to treat. Aside from isolated or predominantly acute right heart failure patients (ESC-6), ESC-1 patients with significant impaired contractile function, markedly dilated heart chambers, and significant dynamic mitral regurgitation with diminished BP and a low tendency to retain fluids, may present “cold and dry”. These patients will probably need inotropes, maybe vasopressors as well, due to hypoperfusion and hypotension, as usually BP will be low rather than high. Treatment of hypoperfusion is essential, diuretics are here second line to those with enlarged hearts [5, 7, 50, 308, 479]. However, if a predominant or isolated right heart failure (ESC-6) is the reason of concern, diuretics (possibly in combination with vasopressors and or inotropes) may be absolutely indicated as, due to acute RV dilation and pericardial constraint, diastolic ventricular interaction is present and effective (overview by Harjola [510])—read more about this issue in Chap. 4.
Patients with ACS complicated by acute heart failure (ESC-5) may show a warm and dry picture, however usually a “warm and wet profile”, sometimes a pre- shock or cardiogenic shock constellation. They represent in any case a high risk group and immediate (<than 2 h after admission) invasive coronary intervention for both, ST elevation myocardial infarction and non–ST-elevation myocardial infarction is mandatory [25, 431, 511, 512]. As up to 70–80% of the patients suffer from multi-vessel (stenosis/occlusion >1 vessel) disease [13, 513–515] even CABG may be necessary. For further details regarding this issue, please read Chap. 3, cardiogenic shock.
2.6.2 Initial Therapeutic Approach
2.6.2.1 Treatment of Underlying Diseases [25, 470, 471, 511, 512, 514]
Primary angioplasty or thrombolysis of acute ST- and non-ST elevation myocardial infarction;
Percutaneous coronary intervention (PCI) in patients suffering from refractory myocardial ischaemia;
Antibiotic treatment for patients with endocarditis;
Pericardiocentesis in order to relieve cardiac tamponade caused by trauma, acute pericarditis, malignancy or other cause;
Treatment of acute arrhythmias (i.e. pacemaker, antiarrhythmic drugs, acute ablation);
Urgent surgical intervention on complications of myocardial infarction or aortic dissection;
Antibiotic treatment for systemic infectious diseases with heart failure as a complication.
2.6.2.2 Common Basic Measures
The patient should also be assessed according to the ABC (airway, breathing, circulation) method of resuscitation, which tends to be standard but with emphasis on particular areas:
The patient should sit upright;
If peripheral O 2 -saturation is <90% (paO2 < 60 mmHg (8.0 kPa) [5, 431, 516] (an ESC class I level C recommendation [5]). A saturation of <90% is an important sign that the patient most probably has pulmonary oedema [481]—these patients should be classified as ‘wet’ [158, 408, 432, 436]. Note: Oxygenation of non-hypoxic patients or even hyperoxygenation can be associated with reduced coronary blood flow, increased systemic resistance (vasoconstriction), reduced cardiac output and shows a trend to higher mortality [517, 518] and should therefore be restricted to hypoxemic patients [431].
Morphine sulphate: 1–3 mg IV, may be given to very anxious and distressed patients, can be repeated several times. Class II a recommendation, Evidence level C [5].
2.6.2.3 Typical and Specific Measures
Diuretics and Ultrafiltration
Loop diuretics are first-line therapy of AHFS [5, 16, 359, 476]. They should, due to usually peripheral congestion, be given preferably intravenously (i.v.) [5, 490].
Diuretics directly reduce excess levels of extracellular fluid [157]. They indirectly exert hemodynamic effects and reduce the LVEDP by venodilation [489], hence promote the relief of symptoms caused by congestion [145, 157]. Loop diuretics given i.v. commence their diuretic effect after approximately 30 min with the venodilating effects commencing already 15 min after administration, and both actions last up to 2 h [506]. Diuretics are indicated in basically all patients with acute left heart failure who show symptoms secondary to congestion and fluid retention/fluid overload [4, 145, 478, 480].
A class I, level B recommendation of the ACCF/AHA [7] and a class I, level C by the latest ESC recommendation from 2016 [431].
Diuretics may produce complications due to reduction of glomerular filtration rate (GFR) [522] and a further activation of the neurohumoral systems [482, 493, 523] with amplification of vasoconstriction, hence a (further) decrease in SV may apply [480]. Unfortunately, there have even been hints that higher dosages of diuretics may increase in-hospital and overall mortality [480–483]. However, by reducing intravascular volume and filling pressures, as well as peripheral and pulmonary congestion [490], diuretics may even blunt neurohormonal activation [484]. The most relevant undesired side effect that diuretics may induce in the acute setting clearly is vasoconstriction [484].
Dosage of furosemide: Start with 20–40 mg i.v. [145, 431, 504], 80 mg if serum creatinine >200 μmol/L [471].
Avoid higher dose boluses (>1 mg/kg) which may induce reflex vasoconstriction [484] and worsen the vascular resistance.
Dosing is still a matter of debate [524, 525]: A Cochrane analysis by Salvador [526] established clues that a continuous infusion of loop diuretics provides a larger diuresis and greater safety than intermittent bolus doses. In contrast, the DOSE-study (Diuretic Optimalization Strategies Evaluation) evaluating i.v. bolus vs. continuous infusion application of loop-diuretics, as well as high dosages (2.5 times the patient’s dose prior to admission, on average 773 mg within 72 h, usually roughly 130 mg every 12 h) vs. low dosages (the same dose the patient was on prior to admission, mean 358 mg within 72 h, usually every 12 h 60 mg) found no superiority of the continuous infusion in either group, but an earlier symptom relieve in the high dose group was seen, probably at cost of a transiently worsened renal function recorded [359]. Furthermore, a number of secondary end points were in favour of a high dose application.
Progressive edema development despite sufficient increased oral or i.v. dosages of diuretics is referred to as diuretic resistance [527]. 20% to 30% of patients with severe LV dysfunction develop diuretic resistance [527]. Therapy-resistance implies a poorer prognosis [528].
To overcome, higher dosages and/or combinations of diuretics, and the avoidance of nephrotoxic agents like NSAID’s are recommended [5, 7, 16]: As such, in patients resistant to diuretic therapy, higher dosages [16, 195, 359] or a combination of diuretics [529–531]) are indicated. The ACCF/AHA suggests a combination of loop-diuretic and another, preferably thiazide, a class IIa, level B evidence [7]. The ESC valuates the combination therapy as a class IIb, level C recommendation [431].
Torasemide (a typical loop diuretic agent [7, 533]) has shown a better functional improvement, a lower incidence of hypokalaemia and a lower mortality [533] when compared to furosemide and other loop diuretics [534]. It produces a lower transcardiac aldosterone gradient due to mineralocorticoid receptor blocking effects [535].
Continuous renal replacement therapy (precisely continuous ultrafiltration—UF) has initially been considered to start up early on in patients with acute severe heart failure, who are fluid overloaded, in order to mitigate symptoms attributed to fluid overload, or who show an inadequate response to diuretic therapy, are oligo-anuric [536–538] and/or have deteriorating renal failure as described by Mehta [482] and others [537, 539]. The Unload Trial (Ultrafiltration vs. Diuretics for Patients Hospitalised for Acute Decompensated Chronic Heart Failure) [539] was the first study showing a superiority in clinical outcomes of the ultrafiltration group compared to the diuretic agent group. Furthermore, very progressively, peripheral venous access and new, small sized ultrafiltration equipment was used. Two further small trials confirmed those results, stating that using peripheral ultrafiltration, more fluid was removed and renal function was not further compromised compared to diuretic therapy [538–540 ].
Applying UF, the negative effects of diuretic drugs can be avoided [472, 482]. Furthermore, it should be stressed that continuous UF exhibited, in fluid overloaded patients, if any at all, only a minimal effect on MAP [537, 539, 541].
Meanwhile, the initial encouraging aspects could not be substantiated and a recently published larger trial did not find UF to be more effective than medical therapy [542, 543]. However, creatinine elevation in itself should not be perceived as a principally worse sign and hint of unfavorable prognosis in ADHF [544], and as such, the big cardiological societies have become far more restrictive and recommend to consider UF only in case of refractory congestion due to diuretic—resistant cases [539, 542, 545] (ACCF/AHA II b, level C [7], ESC class II b, level B [431]) and in severely fluid overloaded patients to cope symptoms [539, 542, 545], rated as a class II b level B by both, the ACCF/AHA [7, 539, 545] and by the ESC [5, 431].
Vasodilators
Nitroglycerin (GTN) may be added to diuretics in all patients as long as the systolic BP > 110 mmHg [5, 7, 546], MAP >60–70 mmHg [481, 536], however may be applied as first line drug in hypertensive individuals [5]. The ESC [431] even recommends vasodilators to be considered as initial therapeutic measure in hypertensive (sBP > 140 mmHg) AHF in accordance to several study results [481, 504, 509, 547], as well a class II a, level B evidence [5, 7, 431, 481].
Namely if applied early on, the ADHERE register found a significant lower in-hospital mortality rate and a shorter length of stay in patients who received vasodilating drugs within the first 6 h after admission compared to those who received them later—indeed, most of them in addition to diuretics [195, 548].
Dosage: 20 μg/min up to 200 μg/min [4, 5, 7]. GTN-resistance can be remedied by increasing doses [549].
In case of phosphodiesterase 5-inhibitor treatment, GTN is contraindicated [550].
Note that even very low doses (<0.5 μg/kg/min) of GTN will decrease the LV wall stress (end-diastolic and end-systolic) with reductions of the aortic (central) blood pressure (direct afterload faced by the ventricle), but without a detectable drop of systemic pressure or perfusion in the periphery (tissue perfusion)—a very welcome and desirable effect [551, 552].
Nitroglycerin, although never evaluated in prospective randomized AHF trails [318], definitely displays, compared to diuretics, a few beneficial effects which potentially should favour GTN to be used as first-line approach: Cotter found a greater effectiveness in controlling severe pulmonary oedema [481], nitrates exhibit a more balanced hemodynamic profile [157, 480] with faster reduction in wall stress and LVEDP without reducing the CO [553], very low dosages diminish ventricular load without the risk of systemic blood pressure drop [551, 552], and there are no significant side effects (predominantly only headache) to be expected [504]. However, unfortunately the beneficial properties GTN shows do not translate into a clinical benefit which has lowered its usage and rating [316, 433, 481, 504, 506–508].
Cause of concern is especially that vasodilators may induce hypotension [84, 504] which is associated with several adverse effects, most important myocardial ischemia [68, 554]. However, a reduction in afterload will, as a rule, lead to an increase in flow (SV/CO), preventing the development of hypotension, thus the MAP will be maintained or may increase but at least should not fall [555–557]. In daily clinical practice, when the peripheral resistance (afterload) is lowered by administration of vasodilating agents, the LV wall stress (end-diastolic and end-systolic) will be reduced [500, 501]. Simultaneously the SV will increase due to the reduction in afterload [407, 439] with an increase in forward flow [496, 497, 556]. Furthermore, particularly in severe dilated heart failure, the reduction in LV outflow resistance and filling pressures leads to a concomitant substantial decrease in mitral regurgitation potentially increasing in SV/CO [131, 493, 496, 497, 502].
However, if, with this approach, the blood pressure cannot be maintained and there is no increase in
SV/CO, one of the following circumstances should be considered and treated:
Nevertheless, if vasodilators are applied, there is some justified risk for blood pressure drops which may have serious adverse effects: Several recently published large studies [30, 35, 49] have all found that a sBP < 120 mmHg is a strong indicator of poor (short term) outcome. Hypotension impairs autoregulation [505, 561–563] and, if persistent, will aggravate any myocardial perfusion deficit [554] and will play a part in a vicious cycle leading to a more and more severe ischaemic myocardium [68], worsening the situation. Therefore caution is recommended in initiating vasodilator therapy or drugs with vasodilative effects (i.e. Dobutamine, Levosimendan) if sBP < 120 mmHg.
As such, although somewhat arbitrary, most authors recommend not to use vasodilators if sBP is below (100-) 110 mmHg [5, 21, 433, 546, 564]. Just to reiterate, the ECS sets sBP lower than 90 mmHg as the limit [431] which is really surprising and not consistent with the literature results, e.g. in the French survey, a sBP of ≥120 mmHg showed a better (short-term—long-term has not been studied) outcome [49], the OPTIMISE—study revealed a significant higher mortality if the sBP was below 120 mmHg [69].
Nitroprusside is a potent venous and arterial vasodilator [565] and is extremely effective in reducing the afterload as well as reducing the pre-load, and thus lowering end-systolic and end-diastolic wall stress [497]. It decreases the neurohumoral activation markedly [566]. In patients where the systolic BP exceeds 120 mmHg, and particularly in hypertensive crises underlying pulmonary edema, the use of nitroprusside should be seriously considered [137], as some authors recommend [4, 565]. A further important indication is severe mitral regurgitation [498, 499].
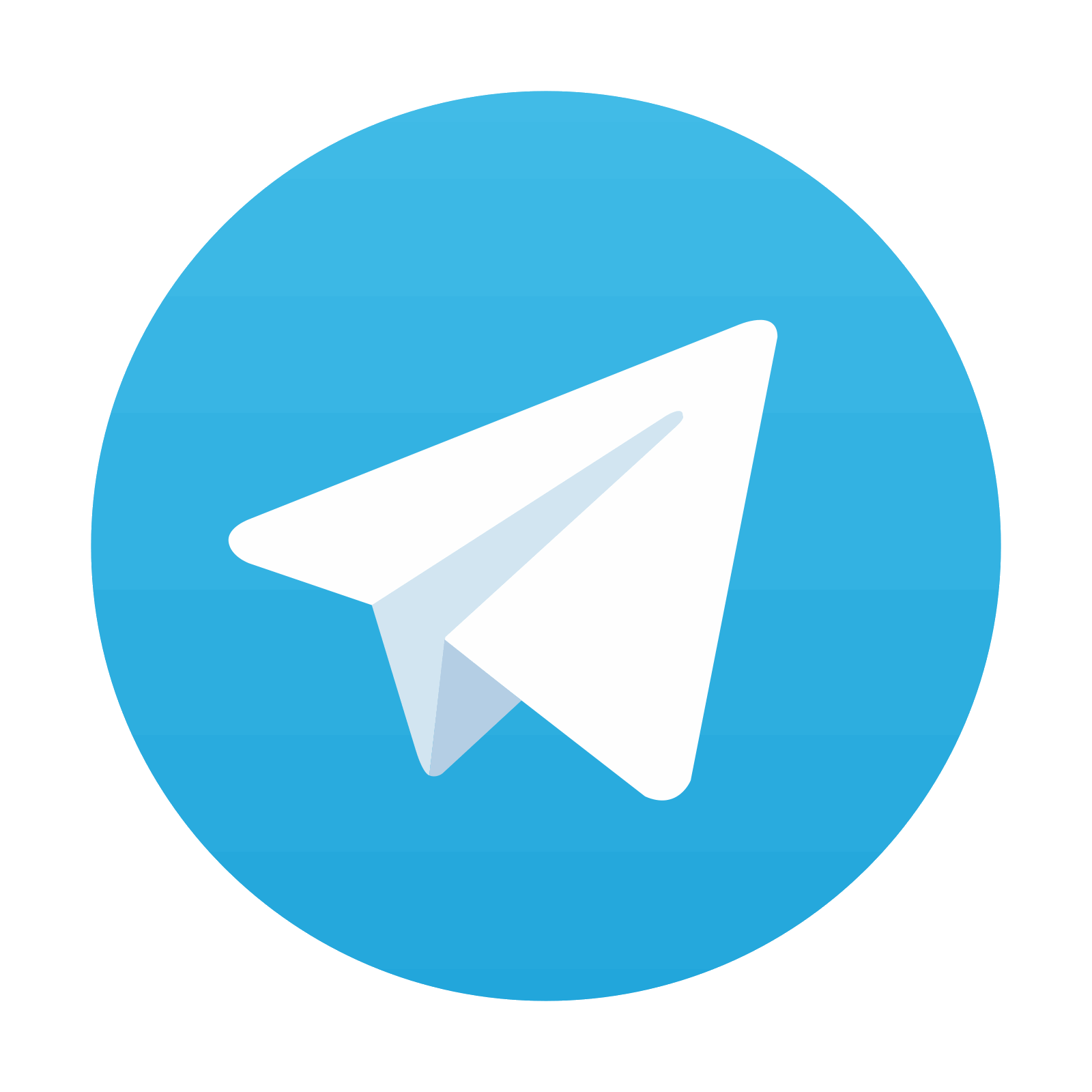
Stay updated, free articles. Join our Telegram channel
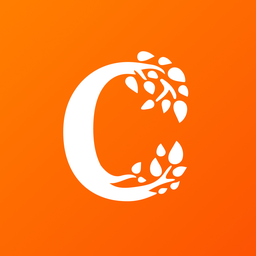
Full access? Get Clinical Tree
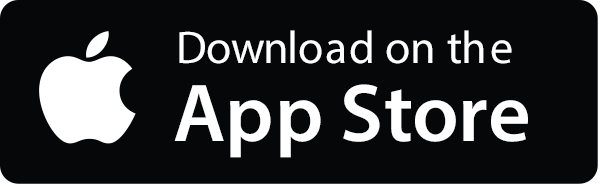
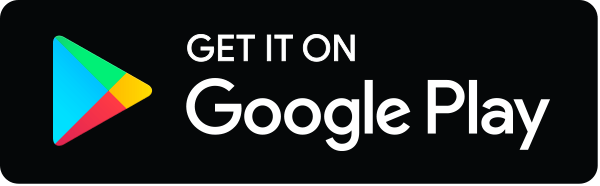
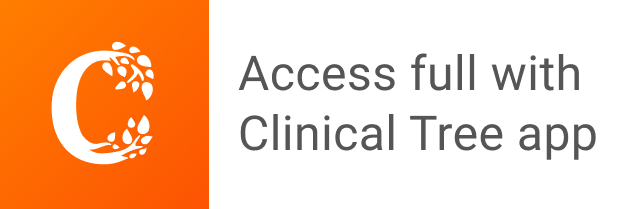