Acclimatization versus adaptation The consequences of exposure to acute hypoxia Factors impacting successful acclimatization Can humans preacclimatize to high altitude? To mountaineers, acclimatization is the process by which they become more comfortable at altitude and find they can perform better than when they first arrived (Houston 1955; Roach 2019). The concept is nicely encapsulated by a statement from Charles Houston (1955), who, following decades of experience climbing at very high altitudes, wrote: “There is an altitude frontier beyond which humans cannot become accustomed to oxygen lack, but the approach to that frontier … is made possible by acclimatization—the process of becoming accustomed to an environment foreign to the organism.” For individuals traveling to high altitude, acclimatization prevents or alleviates symptoms and signs of acute altitude illness and restoration of some of the exercise performance lost on arrival at altitude (Latshang et al. 2013; Subudhi et al. 2014), whereas for physiologists, altitude acclimatization is, strictly, the sum of all the beneficial changes in response to altitude hypoxia that occur with time spent at a given altitude, and that eventually disappear on descent to low altitude. It refers only to the changes in response to hypoxia seen as beneficial as opposed to changes that result in illnesses such as acute mountain sickness (AMS), high altitude cerebral edema (HACE), or high altitude pulmonary edema (HAPE) and are considered pathological. This chapter considers the issue of acclimatization in greater detail. After clarifying the concept of acclimatization, the chapter provides an overview of the major physiological responses to hypoxia, which serves as a preview to the more detailed information in the chapters that follow. We then consider factors affecting the success of acclimatization and how long the beneficial responses last following descent to lower elevation. Because there are elevations above which individuals can no longer acclimatize, the chapter concludes with a discussion of the issue of high altitude deterioration seen at very high altitudes. Acclimatization is often defined by the increase in ventilation and the consequent reduction in PCO2 and increase in PO2. However, these alone are not satisfactory measures since many other processes are involved. The processes of acclimatization all tend to reduce the fall in PO2 as oxygen is transported through the body from the outside air to the tissues. However, even in the best acclimatized individual the tissue PO2 is not restored to the sea level value and performance remains impaired. This is illustrated in Figure 7.1, which shows the inspired and alveolar PO2 (PAO2) of a subject exposed to acute hypoxia and after acclimatization. Also shown are the normal PAO2 at sea level and the arterial PO2 (PaO2) at which continuous oxygen therapy is advised for patients with chronic obstructive pulmonary disease (COPD); even acclimatized subjects are below this level at altitudes above 4000 m. Figure 7.1PAO2 of unacclimatized (acute exposure) and acclimatized subjects at altitudes from 1000 m to 6000 m. Also plotted are the normal sea-level PAO2 of 100 mmHg and the PaO2 at which patients with COPD are entitled to continuous oxygen therapy, 55 mmHg. Note that even acclimatized subjects are below this latter value when above altitudes of about 4000 m. (Source: West 2004.) The major benefits of acclimatization are to protect against acute altitude illness (Subudhi et al. 2014), to improve cognitive performance to near sea-level values (Roach et al. 2014), and to boost submaximal exercise performance (Fulco et al. 2005; Horstman et al. 1980; Latshang et al. 2013; Subudhi et al. 2014) without changing peak oxygen consumption (Beidleman et al. 1997; Calbet et al. 2003a; Calbet et al. 2003b; Calbet et al. 2004; Fulco et al. 2002; Fulco et al. 2005; Lundby et al. 2004; Lundby et al. 2007; Sutton et al. 1988; Wolfel et al. 1991). To understand the effects of acclimatization on exercise, tests of submaximal endurance are more useful than peak oxygen consumption or all-out time trial performance over a short distance, as high altitude travelers rarely perform at these levels of exertion. For example, mountaineers try to preserve energy to sustain efforts across multiple days and might actually put themselves at risk of serious harm, or death, if they truly reached the point of exhaustion. Their ability to cover more ground faster while preserving a functional reserve is a hallmark of acclimatization supported by anecdotal accounts (Houston 2005; Messner 1989). Despite this, the physiology behind the improvement in sustained, self-regulated submaximal performance at altitude remains unexplored. The transient phenomenon of acclimatization must be distinguished from the concept of adaptation, which refers to characteristics present in long-term high altitude residents that have arisen as a result of natural selection working on the gene pool. These characteristics serve to distinguish people born and bred at high altitude from even well-acclimatized lowlanders. There is debate about whether these characteristics are due to environmental factors operating during early growth or due to genetic causes. Recent genetic evidence points to adaptation happening in people resident at high altitude for generations in South America, Tibet, and Ethiopia (Chapter 4). The remainder of this chapter will focus on the issue of acclimatization while issues related to adaptation are discussed in Chapters 4 and 6. To further explore the process of human adjustment to hypoxia, one must turn to an understanding of the oxygen transport system in an oxygen-limited environment. The response of the body to hypoxia depends crucially on the rate of onset as well as the degree of hypoxia. For instance, the effect on a pilot of sudden loss of oxygen supply in an unpressurized aircraft at the height of the summit of Mount Everest is quite different from the effect of similar altitude on a climber who has spent some weeks at altitude. The pilot would probably lose consciousness in a few minutes (Figure 7.2), whereas the climber, though very breathless, will not only remain conscious but also be able to perform meaningful physical work. The difference in outcomes between the pilot and the climber is due to the process of acclimatization. Figure 7.2The effect of sudden exposure to various altitudes. At extreme altitude, consciousness will be lost after an average time indicated by the curve on the right. There is considerable variability in this time. (Source: Pickard and Gradwell 2008.) The effect of acute, often severe, hypoxia has been studied intensively since Paul Bert first pointed out the danger of ascent to high altitude to early balloonists (Chapter 1). Figure 7.2 shows the effect of sudden exposure to various increasing altitudes. At modest altitude, breathlessness may be felt on exertion and some rise in heart rate noticed, but the main effect is on the central nervous system. At 4000–5000 m some tingling of the fingers and mouth may be noticed, but although the subject would now definitely be hypoxemic, there is very little subjective sensation to indicate this fact. Above about 5000 m, some subjects may become unconscious, and above 7000 m, most will be so (Pickard and Gradwell 2008). There is considerable individual variation in response to acute hypoxia. From Figure 7.2, we can see that, on acute exposure to the altitude equivalent to the summit of Everest, the unacclimatized subject remains conscious for only about two minutes. Figure 7.3 shows the oxygen transport system at sea level and at high altitude. This diagram can be used as a “table of contents” of changes resulting from acclimatization and serves as a preview of more detailed discussions of the physiologic responses to hypoxia in the chapters that follow. PO2 falls at each stage as oxygen is transported from ambient air to inspired air (PIO2) to the alveolar space (PAO2), arterial blood (PaO2), and mixed venous blood ( Figure 7.3The oxygen transport system from outside air through the body at sea level and at an altitude of 5800 m. PB, barometric pressure; Δ, rest; ●, maximum exercise. (Adapted from Pugh 1964.) The ambient PO2 of dry air at sea level is about 160 mmHg (20.9% of 760 mmHg the barometric pressure). At an altitude of 5800 m, in the example shown in Figure 7.3, the barometric pressure is just half that at sea level (Chapter 2), so the ambient PO2 is also half the sea-level value, 80 mmHg. The drop seen in the figure from ambient to inspired PO2 of about 10 mmHg is due to the addition of water vapor to the inspired air as it is humidified and warmed to body temperature in the nose, mouth, larynx, and trachea. The water vapor pressure at body temperature is 47 mmHg, and this displaces almost 10 mmHg PO2. This physical cause of PO2 reduction is beyond the control of the body and so applies equally at high altitude, although its effect is proportionately more important there. At sea level there is a drop of about 50 mmHg at this point in the oxygen transport cascade. This drop can be thought of as being due to the addition of carbon dioxide and the uptake of oxygen and so depends, in part, on the metabolic rate. However, it also depends on alveolar ventilation and for a given oxygen uptake and carbon dioxide output the size of this reduction is entirely due to ventilation. A doubling of ventilation results in a halving of this drop. If ventilation were infinite there would be no reduction and alveolar gas would be fresh air. After acclimatization at 5800 m, resting alveolar ventilation is approximately doubled and this step in the system, as shown in Figure 7.3, is halved. This increase in ventilation is one of the most important aspects of acclimatization and the changes in control of breathing responsible for it are discussed further in Chapter 9. Figure 7.3 also shows the effect of exercise on the oxygen transport system (dashed lines). At sea level, the PAO2 is little changed by exercise but at altitude the increase of ventilation is far greater in response to exercise than at sea level, so that with exercise the PAO2 is increased and PACO2 is decreased (Chapter 18). Oxygen diffuses across the alveolar-capillary membrane, resulting in a small pressure drop but in the normal lung this accounts for less than 1 mmHg. The total alveolar-arterial oxygen difference ([A-a]ΔO2) at sea level is 6–10 mmHg, with the bulk of this difference resulting from ventilation/perfusion (V/Q) inequality, due to the fact that even in the healthy lung, the matching of ventilation to blood flow is not perfect. At altitude, at rest, the normal (A-a)ΔO2 is slightly lower than the sea level value because the arterial PO2 falls on the steep portion of the hemoglobin-oxygen dissociation curve (see Chapter 8 for a more detailed discussion) (Sutton et al. 1988). Ventilation-perfusion inequality is modestly reduced, because of the increase in pulmonary artery pressure due to hypoxia (Chapter 11), reducing the gravitational effect on the distribution of blood flow in the lung. This was thought not to increase the diffusing capacity of the lung during acclimatization (West 1962), although several studies have demonstrated an increase in the diffusing capacity for carbon monoxide (Agostoni et al. 2011; Dehnert et al. 2010). On exercise at high altitude, the (A-a)ΔO2 increases significantly due to diffusion impairment and increasing ventilation-perfusion inequality (Wagner et al. 1987) and becomes important in limiting exercise performance. This is shown as the dashed line in Figure 7.3. The issue of diffusion limitation and other aspects of gas exchange at high altitude are explored more fully in Chapter 8. The last drop in PO2 shown in Figure 7.3 from arterial to mixed venous blood is due to the uptake of oxygen in the systemic capillaries. Its magnitude is influenced by the metabolic rate, the cardiac output, and the oxygen carrying capacity of the blood, i.e., the hemoglobin concentration ([Hb]). A modest increase in [Hb] is probably beneficial in that it increases the oxygen carrying capacity of the blood and, at altitudes up to about 5000 m, this is sufficient to balance the reduction in oxygen saturation due to reduced PaO2 and restore the oxygen content of arterial blood to sea level values (though now at a lower PO2) (Calbet et al. 2003a). With moderate, physiological increases in [Hb] there seems to be a close reciprocal relationship between [Hb] and blood flow so that oxygen delivery to particular organs (e.g., working muscles or brain), remains constant over a range of [Hb] and The in vitro hemoglobin-oxygen dissociation curve is shifted slightly to the right at moderate altitudes due to an increase in 2,3-diphosphoglycerate. However, the position of the in vivo curve is uncertain because of uncertainties about the in vivo pH (Winslow and Monge 1987). There is probably some degree of respiratory alkalosis that offsets the effect of the 2,3-diphosphoglycerate. At extreme altitude, above about 7000 m, there is quite severe respiratory alkalosis and therefore a definite leftward shift in the dissociation curve. This is beneficial to the climber at least as far as oxygen delivery to working muscles is concerned. This is because more oxygen is loaded into the blood in the lungs while the unloading of oxygen in the muscles is only slightly reduced. This is because the difference in position of the curves for normal and higher pH, at very low PO2, is so small. (See Chapter 10 for a fuller discussion of the position of the oxygen dissociation curve and the graphical representation, Figure 10.1.) Cardiac output increases with acute hypoxia but decreases as [Hb] increases with continued stay at altitude over the first one to two weeks. These changes, as well as other effects on the vascular system, are discussed in Chapter 11. At the tissue level there is an increase in the density of capillaries due to a reduction in diameter of muscle fibers (Chapter 14). Taking the body as a whole, the mixed venous PO2 can be thought of as reflecting the mean tissue PO2. It can be seen in Figure 7.3 that the effect of these processes of acclimatization is to maintain this critical PO2 as near as possible to the sea-level value. Beyond this, there is the possibility of adjustments at the tissue level involving the microcirculation and then intracellular mechanisms. These form the subject of Chapter 14. No objective test can tell a mountaineer or other high altitude traveler if they are fully or only partially acclimatized. In practice, this is not a serious limitation because the positive benefits of transitioning from unacclimatized to acclimatized are readily apparent (Roach 2019). For the acclimatized individual at a given altitude, tasks of daily living become easier to accomplish, tolerance of low to moderate intensity exertion markedly improves, and the symptoms of AMS, if present initially, fade. The investigation of the underlying molecular and physiological mechanisms driving acclimatization has been largely stagnant until recently. For example, research into the pathophysiology of AMS often uses models of cross-over control conditions at sea level or comparisons of those with and without AMS at high altitude, or, even worse, a cross-over design where individual variation is ignored. Only recently have studies begun to compare the unacclimatized AMS subject to themselves when they become acclimatized (Subudhi et al. 2014). As pointed out more than 60 years ago by Charles Houston, the acclimatized climber is largely protected from the challenges of acute hypoxia (Houston 1955). Determining how this happens and if it can be artificially induced holds promise to advance our understanding of the mechanisms driving acclimatization and AMS. The following is a summary of the science regarding aspects supporting successful acclimatization. Because hard data are notably absent in this area, much of the discussion necessarily relies on anecdotal evidence and common sense. The changes involved in acclimatization occur in various systems and with varying time courses. These are illustrated in Figure 7.4, which shows the futility of the frequently asked question, “How long does it take to become acclimatized?” Figure 7.4Time course of various acclimatization and adaptive changes plotted on a log time scale. The curve of each response denoting the rate of change is fast at first, then tails off. From bottom to top, the included variables are heart rate, hyperventilation and hypoventilation, the carbon dioxide ventilatory response (CO2 VR), hemoglobin concentration ([Hb]), changes in capillary density (Cap. Dens.), hypoxic ventilatory response (HVR), and pulmonary hypoxic pressor response (PHPR). Of these changes, the most important are the responses in the cardiorespiratory system and the blood, which occur over the course of days or a few weeks. Figure 7.5 shows the changes in a number of physiological systems over the first 10 days of altitude 4300 m (Muza et al. 2010). Lundby et al. (2004) studied early changes in acclimatization in six lowlanders. Subjects were studied at sea level (normoxia and acute hypoxia) and after two and eight weeks of acclimatization at 4100 m in Bolivia. Local high altitude residents were also studied at altitude. Some results from this study are shown in Figure 7.6 for rest (Figure 7.6a) and maximum exercise (Figure 7.6b). It can be seen that the hematocrit rises during the first two weeks to close to the high altitude residents’ value and is no higher at eight weeks. The PaO2 continues to rise for eight weeks and is still below that of the high altitude residents. Changes in PaCO2 appear to be complete at two weeks while the (A-a)ΔO2 rises with acute hypoxia, is greater at two weeks, and falls slightly at eight weeks. The situation at maximum work rate is similar except that the PaCO2 continues to fall due to increased exercise ventilation. Subudhi et al. (2014) studied 21 college students at sea level and after 16 days at 5200 m in Bolivia; results showed a similar time course for acclimatization. The elevations in arterial oxygenation and [Hb] from day 1 to 16 at 5200 m were similar to those measured in individuals acclimatized for at least 10 days at altitudes ranging from 3800 m to 5260 m (Bebout et al. 1989; Lundby et al. 2004; Wagner et al. 1987; Wagner et al. 2002). Thus, it seems that 10–16 days at 4000–5000 m results in significant acclimatization, and that this duration of exposure can be effective to test acclimatization and its subsequent retention. Figure 7.5Changes in cognitive performance, resting SaO2, exercise SaO2, physical performance, exercise heart rate, and incidence of acute mountain sickness (AMS) in lowlanders ascending directly to 4300 m. (Source: Muza et al. 2010.) Figure 7.6Changes in PaO2, PaCO2, (A-a)PO2, and hematocrit (Hct) at sea level (SL) under normoxia and acute hypoxia, at altitude (4100 m) after two weeks (2W) and eight weeks (8W) of acclimatization, mean results in six lowlanders and eight Amarya high altitude natives (HANat); at rest (a) and maximum exercise (b). (Drawn using data from Lundby et al. 2004.) Surprisingly, when subjects descended for one to three weeks to 1500 m, they experienced neocytolysis (Ryan et al. 2014), a destruction of red blood cells that led to a 13% drop in hemoglobin concentration and thus in arterial oxygen content (CaO2
Introduction
Acclimatization versus Adaptation
The Consequences of Exposure to Acute Hypoxia
The Oxygen Transport System
Ambient to inspired po2
Inspired to alveolar po2
Alveolar to arterial po2
Arterial to mixed venous po2
Factors Impacting Successful Acclimatization
Rate of acclimatization
Stay updated, free articles. Join our Telegram channel
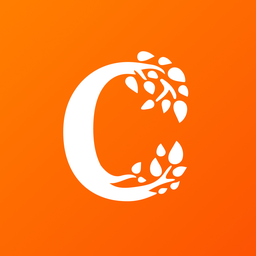
Full access? Get Clinical Tree
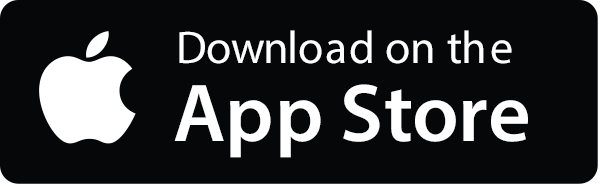
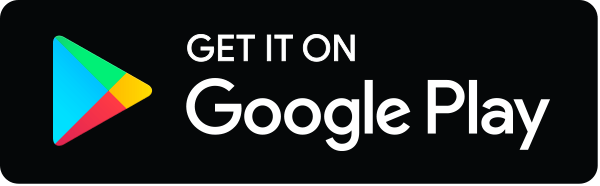