June 2010 marks the 50th anniversary of the first successful human cardiac pacemaker implantation in the United States. On June 6, 1960, in Buffalo, New York, Dr. William Chardack implanted a pacemaker, designed and built by Wilson Greatbatch, an electrical engineer and inventor, in a 77-year old man with complete atrioventricular block, extending the patient’s life by 18 months. This landmark event ushered in a new era of implantable cardiac pacemakers with batteries and leads of high reliability and increasing durability. Over the past half century, the field of electrophysiology and implantable devices for the management of cardiac conduction disturbances has evolved dramatically. Today’s pacemakers include increasingly complex features such as telemetry monitoring, autoprogrammability, and hemodynamic sensors. New-generation leads present a sophisticated design with improved geometry and steroid-eluting tips to reduce chronic inflammation, maintaining a low pacing threshold and high sensing capability. The lithium iodide battery remains the mainstay of implantable pacemaker systems, exhibiting a multiple-year lifespan, slow terminal decay, and a reduced size and cost of production. Although Greatbatch’s first successful pacemaker implantation remains a seminal scientific contribution to modern cardiovascular disease management, emerging developments in this field may challenge its preeminence. Important challenges such as imaging compatibility, lead durability, and infection prevention are being addressed. Novel concepts such as leadless and biologic pacing are under active investigation. In conclusion, Greatbatch’s historic achievement 50 years ago reminds us that technologic progress is timeless, as efforts to enhance clinical outcomes and the quality of life continue unimpeded into the 21st century.
The era of practical human cardiac pacemaker implantation began more than half a century ago in an unlikely place. In 1956, in his laboratory at the University at Buffalo, New York, an electrical engineer, Wilson Greatbatch, made a remarkable and fortuitous discovery. Assisting the nearby Chronic Disease Research Institute as a newly appointed assistant professor of engineering, he was assembling a marker oscillator designed to record fast heart sounds. While searching for a 10,000-Ω resistor to fit the circuit, he reached into his resistor box and mistakenly grabbed a similarly colored device with a much higher resistance of 1 MΩ. Unknowingly, he plugged in the incorrect resistor, and the oscillator immediately started to generate a repetitive 1.8-ms pulse, followed by a 1-second pause interval. Having always been fascinated by the medical field, he recognized that this pulse rate was similar to that of the human heart. He also recalled a conversation a few years earlier with 2 visiting surgeons regarding the therapeutic challenge posed by atrioventricular (AV) block and the inability to effectively treat this condition. Greatbatch quickly realized that his serendipitous “mistake” had just resulted in new circuitry that could potentially control the human heartbeat. Over the next 2 years, with a mere $2,000 budget funded from personal savings, Greatbatch refined his device, manufacturing in his backyard workshop ( Figure 1 ) 50 handmade implantable pacemakers, each consisting of his miniature pulse generator powered by mercury zinc batteries and encased in epoxy resin and silicone rubber.
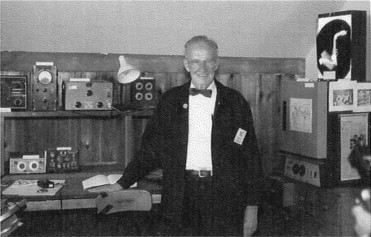
The First Pacemaker Implantation in June 1960
The year 2010 marks the 50th anniversary of the first successful human cardiac pacemaker implantation in the United States, which took place at the Millard Fillmore Hospital in Buffalo, New York, on June 6, 1960, after two years of pre-clinical work conducted with Dr. Andrew Gage at the Buffalo Veterans Affairs Hospital ( Figure 2 ) . Dr. William Chardack, chief of surgery at the Buffalo Veterans Affairs Hospital, first implanted Greatbatch’s pacemaker generator into a 77-year-old man with complete AV block ( Figure 3 ) . The patient had symptomatic complete AV block, with syncopal spells and falls that resulted in several injuries. A bipolar epimyocardial electrode had been surgically implanted 6 weeks earlier, externalized, and connected to an external pacing device until the myocardial stimulation threshold had stabilized at 3 mA. During the operation of June 6, the externalized lead was then surgically sterilized in the operating room, connected to the new Greatbatch pacemaker generator, and inserted into a subcutaneous pocket in the patient’s abdomen.
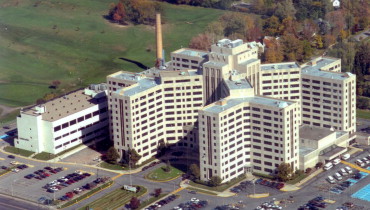
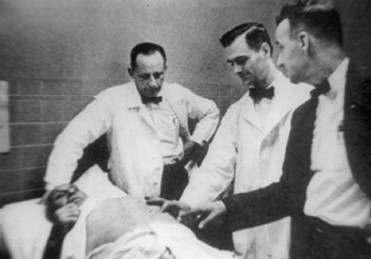
The original pacemaker generator measured 6 cm in diameter and 1.5 cm in thickness and consisted of just 8 components, including pulse-generating circuitry linking 2 transistors, and mercury zinc batteries of 1.35 V ( Figure 4 ). Its hardwired output was a 1-ms biphasic pulse of 8 V into the myocardium every second. This newly implanted device extended the patient’s life by 18 months and was widely heralded as a milestone in pacemaker development. Greatbatch’s pioneering work surmounted a major hurdle by his developing the first practical and reliable permanent implantable cardiac pacemaker. The first report of this device implantation was published in the October 1960 issue of Surgery ( Figure 5 ).
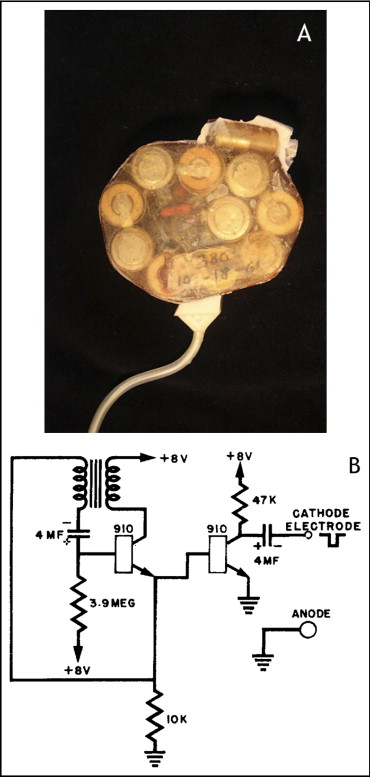
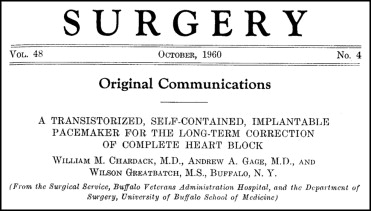
Early Historical Attempts to Achieve Effective Human Pacing
The first pacemaker-like device was developed in the early 1930s by New York cardiologist Albert S. Hyman as a means to restart the human heart. Dr. Hyman, along with his brother, designed a portable machine known as the “Hyman Otor” that used a manual crank generator coupled with a current-interrupting device to deliver an electrical current, which was then conducted down a long needle into the right auricle of the heart, rhythmically stimulating it until it restarted. In the early 1950s, Boston cardiologist Paul M. Zoll developed an external pacemaker. Although it was a major technologic advance, the device proved impractical for long-term use because the high current required for pacing caused skin irritation and significant discomfort, requiring sedation of the patient.
In the mid-1950s, the pioneering cardiac surgeon C. Walton Lillehei identified AV block as an important cause of postoperative death after otherwise successful surgical correction of congenital heart disease. In collaboration with physiologist John A. Johnson and surgical resident Vincent L. Gott, he devised a system for temporary cardiac pacing using epimyocardial wires connected to a Grass stimulator. At the request of Dr. Lillehei, the first portable transistorized battery-powered external pacemaker was developed in 1957 by the engineer Earl E. Bakken. The basic design of this device is still in use today.
The first self-contained, fully implantable pacemaker was inserted in a human in Stockholm, Sweden, on October 8, 1958. Arne Larsson, who had complete AV block and had severe Stokes-Adams attacks, was the first recipient. The pacemaker was implanted by surgeon Åke Senning, using an open thoracotomy and a device designed by Rune Elmqvist. This implantable pacemaker used a nickel cadmium battery, which required frequent transcutaneous recharging. The first device lasted only 3 hours, and a second pacemaker was implanted the following day. The second device also failed soon thereafter. The patient subsequently underwent another 21 pacemaker generator implantations throughout his lifetime. Although this operation represented a landmark in the history of cardiac pacing, the limited battery capacity and durability of the Elmqvist-Senning pacemaker was a significant impediment to its clinical adoption. In contrast, the novel Greatbatch design used mercury zinc batteries and had less current drain, allowing the self-contained power source to last for several years. This critical technologic improvement in the endurance and reliability of the implantable pacemaker device allowed widespread clinical application of this new therapy.
Early Historical Attempts to Achieve Effective Human Pacing
The first pacemaker-like device was developed in the early 1930s by New York cardiologist Albert S. Hyman as a means to restart the human heart. Dr. Hyman, along with his brother, designed a portable machine known as the “Hyman Otor” that used a manual crank generator coupled with a current-interrupting device to deliver an electrical current, which was then conducted down a long needle into the right auricle of the heart, rhythmically stimulating it until it restarted. In the early 1950s, Boston cardiologist Paul M. Zoll developed an external pacemaker. Although it was a major technologic advance, the device proved impractical for long-term use because the high current required for pacing caused skin irritation and significant discomfort, requiring sedation of the patient.
In the mid-1950s, the pioneering cardiac surgeon C. Walton Lillehei identified AV block as an important cause of postoperative death after otherwise successful surgical correction of congenital heart disease. In collaboration with physiologist John A. Johnson and surgical resident Vincent L. Gott, he devised a system for temporary cardiac pacing using epimyocardial wires connected to a Grass stimulator. At the request of Dr. Lillehei, the first portable transistorized battery-powered external pacemaker was developed in 1957 by the engineer Earl E. Bakken. The basic design of this device is still in use today.
The first self-contained, fully implantable pacemaker was inserted in a human in Stockholm, Sweden, on October 8, 1958. Arne Larsson, who had complete AV block and had severe Stokes-Adams attacks, was the first recipient. The pacemaker was implanted by surgeon Åke Senning, using an open thoracotomy and a device designed by Rune Elmqvist. This implantable pacemaker used a nickel cadmium battery, which required frequent transcutaneous recharging. The first device lasted only 3 hours, and a second pacemaker was implanted the following day. The second device also failed soon thereafter. The patient subsequently underwent another 21 pacemaker generator implantations throughout his lifetime. Although this operation represented a landmark in the history of cardiac pacing, the limited battery capacity and durability of the Elmqvist-Senning pacemaker was a significant impediment to its clinical adoption. In contrast, the novel Greatbatch design used mercury zinc batteries and had less current drain, allowing the self-contained power source to last for several years. This critical technologic improvement in the endurance and reliability of the implantable pacemaker device allowed widespread clinical application of this new therapy.
Additional Technologic Advances
Batteries
The chief impediment to developing a practical and reliable implantable pacemaker was not the technical pacing circuitry but rather the more basic issue of a sustainable power source. Without the development of a power supply that matched the sophistication and miniaturization of the pacing circuitry, the practical application of the implantable pacemaker could not have occurred. Although the mercury zinc battery introduced in the Greatbatch-Chardack device was superior to the nickel cadmium battery of the Elmqvist-Senning device, it still had limitations. Although a 1- to 5-year lifespan represented significant technical improvement, the reliability of the power source was still insufficient to make the device safe for routine implantation. Because the terminal voltage did not change significantly until the end of useful battery life, the durability of these early implanted devices was also unpredictable. Last, this system produced hydrogen gas and could not be hermetically sealed, leading to the accumulation of water vapor with resultant component failure.
To ameliorate these limitations, extensive research was pursued by multiple investigators, including the development of nuclear-powered pacemakers in the 1960s. In fact, 1 of the first successful implantable pacemakers developed by Chardack and Greatbatch was powered by plutonium, lasting for up to 30 years. The problems inherent in using nuclear-powered pacemakers were the inconvenience met during patient travels and the safe disposal of used cells. Importantly, plutonium was also known to be extremely toxic and potentially fatal at very low concentrations, thus making it impractical for commercialization.
Eventually, lithium-iodine batteries, first developed in 1968 by Catalyst Research Group (Baltimore, Maryland), became the mainstream technology when this battery system was licensed to and refined by Greatbatch in 1973 to power pacemakers. Lithium batteries are suitable for implantable pacemakers because they meet the requirements of long life, low current drain, and proper voltage characteristics. The shelf life of primary lithium cells is typically equivalent to a 10% loss of capacity over 5 years, compared to a similar loss for alkaline cells over only 1 year. Lithium systems are kinetically stable and hence produce no gas; thus, they can be hermetically sealed. In addition, the terminal voltage decay is highly predictable, decreasing slowly enough for end of battery life to be anticipated during routine follow-up. With these optimal characteristics, including a potential life span in excess of 10 years, lower production costs, and smaller size, the lithium-iodine cell became the battery of choice for pacemaker manufacturers.
Leads
From the initial era of bare stainless steel metal wires placed through the chest wall into the left ventricular epimyocardium via thoracotomy, current implantable pacemaker leads have significantly improved quality and reliability.
The limitations of older generation leads included a high fracture rate, dislodgement or displacement, electromagnetic and myopotential oversensing, and inflammation at the electrode tip with increasing thresholds, leading to premature battery drain. Many of these problems have been mitigated, and the development of the optimal pacing lead has continued to evolve and improve over time.
A variety of materials have been used in pacemaker electrodes. After exhibiting unreliable corrosion resistance, bare-metal electrodes were discontinued in the 1960s in favor of porous electrodes with a lower tendency to dislodge or exhibit large increases in capture threshold. Elgiloy, an alloy of cobalt, chromium, and nickel, was discontinued commercially in the 1980s after revealing inferior threshold, polarization, and corrosion properties. In recent years, titanium, iridium, and vitreous carbon (in a variety of combinations) have been in use as electrodes, demonstrating low pacing thresholds with minimal polarization losses. The conductor coils are of a unifilar, multifilar, or cable design to improve tensile strength and increase resistance to fatigue. The currently available bipolar lead systems have coaxial or coradial filaments separated by layers of insulation material.
The ideal electrode tip allows optimal impedance with low and stable pacing thresholds. High pacing impedance is desirable because it requires lower energy for pacing, thus improving the longevity of the pulse generator and minimizing size. Impedance is inversely related to electrode surface area; therefore, to have high pacing impedance, a small surface area is preferred. In contrast, small surface area results in high sensing impedance and could result in excessive attenuation of the cardiac signal. Various studies have identified the optimal cathode surface area as 8 to 12 mm 2 for polished electrodes. Although early models used a round spherical shape with a smooth metal surface, recent models have used a more irregular, textured surface to increase the surface area using microscopic pores, coatings of microspheres, and wire filament mesh. This design allows a low geometric outer area to keep the pacing impedance high but provides an increased surface area to maintain low sensing impedance.
Chronic threshold increase was another issue that faced the developers of pacemaker leads. Several designs, including porous platinum and activated vitreous carbon electrodes, were developed; however, major advancement was not made until the steroid-eluting cathode was introduced by Stokes and Timmis in 1983. The original design was an 8-mm 2 porous platinum-coated titanium electrode that contained a silicone rubber, dexamethasone controlled-release device in an internal chamber. There have been multiple comparative studies supporting the benefit of steroid-eluting electrodes in achieving low chronic pacing thresholds. Current steroid-eluting electrodes approach ideal performance with low stable thresholds of <0.8 V at 0.3 ms maximum for the 98th-percentile patient, high pacing impedance of about 600 to 1,500 Ω, and low source impedance of <1,000 Ω.
The earliest leads used Teflon or polyethylene insulation, which was soon replaced by silicone and polyurethane. Silicone rubber is optimal for its safety, inertness, and biostability but has low tensile, elongation, and tear strength. Minimizing silicone insulation failure required increasing insulation thickness. Tougher silicone, introduced in the 1980s, partially resolved these deficiencies and improved tensile, elongation, and tear strength. Polyurethane, a polymer introduced in late 1970s, exhibited much higher tensile strength and higher elongation and tear strength than silicone, allowing the insulation to be thinner without compromising safety. Polyurethane experienced a setback when degradation and stress-cracking problems were identified in some materials, but the mechanism of degradation was discovered and mitigated, resulting in very low subsequent incidence of metallic ion oxidation failure. Stress cracking has been ameliorated by using stress relieving (annealing) processes and the use of thicker insulation. Preliminary studies suggest that a hybrid coating of silicone and polyurethane may offer improved durability. However, short-term clinical trials have not always predicted long-term performance, and careful monitoring of new lead designs is warranted.
The development of improved fixation mechanisms has dramatically lowered the incidence of lead dislodgment. Lead fixation may be active, through the use of a deployed helix, or passive, through tines that become ensnared in myocardial trabeculations. Although equivalent to passive fixation in long-term stability, active fixation leads allow more choices in implant site selection and may be more amenable to lead extraction.
Lead polarity has been a topic of debate for >40 years. Bipolar leads are generally more desirable, because this configuration minimizes risk for extracardiac stimulation, myopotential oversensing, far-field signals, and electromagnetic interference (EMI). Bipolar leads, however, were initially more complex and prone to higher fracture rates. More recently, reliability has improved and became comparable to unipolar leads. In addition to the improvement of bipolar lead design, the pulse generator has been modified to include software capable of detecting lead fracture (which causes very high pacing impedance) and switching from bipolar to unipolar pacing configuration. With low fracture rates and improved safety features, bipolar leads appear to be preferable in most aspects and have become standard in nearly all endocardial pacing systems.
As summarized previously, pacemaker lead design has improved considerably since the early decades of cardiac pacing, when high fracture rates, dislodgement, EMI, and high capture thresholds were commonplace. The current standard leads are bipolar, porous platinum surfaced, and steroid eluting, with a cathode of 5 to 12 mm 2 and silicone or polyurethane-insulated platinum and iridium or titanium conducting coils. The safety and reliability of these leads generally exhibit 97% to 99% 5-year survival, and the industry continues to modify and improve designs, working for the ideal lead with 100% reliability.
Programmability
Pacemaker programmability can be defined as the noninvasive, stable modification of implanted pulse generator function. The need for rate adjustment was considered as early as the Bakken-Lillehei external pulse generator of 1957, which had a dial for rate adjustment and another to change electrical output. Programmability of permanent pacemakers was introduced in the 1960s with the installation of 2 insulated potentiometers at the lateral edge of the pulse generator that were accessed using special needles inserted percutaneously to adjust rate and output. Alternatively, some pulse generators had magnetic bistable switches. Magnet movement in either direction caused the rate to change from 70 to 100 beats/min, depending on patient preference.
A simple but elegant system of magnetic actuation was introduced in 1972, which varied the pulse duration at a fixed output voltage to change the output per stimulus. This was accomplished by noninvasively rotating a gear train attached to small bar magnets within the implanted pulse generator. The programmer was an external device in which larger bar magnets were manually rotated. The external magnets attracted and caused the rotation of the smaller internal magnets and thus the gear train within the implanted pulse generator. At about the same time, the Cordis Corporation introduced a truly programmable system in which the external programmer introduced a rapid magnetic pulse train. The duration of the train was different for each setting to be programmed. Within the hermetically sealed implanted digital circuit, a reed switch was opened and closed magnetically and counted.
With the introduction of radiofrequency programming and external telemetry, which is now incorporated in all implantable devices, the retrieval and storage of real-time information on battery status, impedance, current, amplitude, and pulse duration, along with other diagnostic information, has become commonplace. However, there is no industry standard used for radiofrequency signals, which vary among the device companies, preventing interchangeable use of programmers. Recently, the collection of telemetry has gravitated toward “remote” and WiFi capability, which eliminates the need to maintain the programmer head over the pulse generator for the duration of interrogation.
“Autoprogrammability” refers to a pacemaker’s ability to alter, and ideally optimize, an individual programmable parameter on the basis of preprogrammed criteria and clinical information collected while in use. This concept has been applied with increasing success to numerous programmable features, including mode-switching algorithms, adjustment of sensing and pacing thresholds, switch to backup safety programming, and adjustment of rate responsiveness. Further development of autoprogrammability is likely to be seen in future pacemaker models.
Rate adaptation
The physiologic understanding central to the development of rate modulation sensors was established by the pioneering hemodynamic studies by Benchimol et al, Karlof, and Samet et al. They validated the benefit of atrial synchrony and rate modulation on cardiac output, demonstrating that with exercise, an increase in heart rate is necessary to maintain optimal cardiac output and hemodynamics.
The concept of sensor-driven pacing was first described in the 1970s. Minute ventilation, QT-interval sensors, and accelerometers were added to the pacemaker platform with the introduction of piezoelectric crystal-based technology and quickly embraced by clinicians. Although a number of other sensors have been tried clinically, only 2, accelerometers and minute ventilation sensors, are widely used in current pacemaker models. A newer sensor uses small changes in right ventricular contraction dynamics to modulate lower rate, a process that appears to be sensitive to mental, emotional, and autonomic stress. More recently, sensors have been combined to achieve an even more effective replication of normal sinus node response to physiologic demands.
Cardiac resynchronization therapy (CRT)
The development of CRT was another landmark event in the use of implantable pacemakers, marking a new era for heart failure therapy. Since its initial description in the early 1990s, multiple clinical trials have supported its use in selected heart failure populations with left ventricular dysfunction, prolonged QRS duration, and moderate to severe heart failure symptoms despite medical therapy. After several early studies that revealed clear improvement in exercise performance, symptoms, and quality of life, large randomized controlled trials demonstrated that CRT, either with or without a defibrillator component, reduced hospitalizations and all-cause mortality in properly selected patients with systolic heart failure and evidence of ventricular dyssynchrony.
With progressive congestive heart failure, electrical dyssynchrony (especially left bundle branch block) leads to progressively uncoordinated and inefficient left ventricular contraction (mechanical dyssynchrony). Hemodynamics may be further worsened with prolonged AV conduction, leading to suboptimal atrial contribution to left ventricular filling. The essential concept underlying CRT is to improve atriobiventricular electromechanical synchrony by multisite ventricular pacing. In a typical CRT device, 3 leads are implanted: standard right atrial and right ventricular leads, along with an additional lead in the coronary sinus directed to a lateral tributary vein. With improvements in sheath and coronary sinus lead design, >90% of patients will have stable placement of suitable coronary sinus leads. When CRT cannot be obtained from the standard endocardial approach, an epicardial lead may be implanted surgically, often using a minimally invasive approach.
With a recent study indicating the benefit of CRT in the prevention of heart failure events, the use of CRT may expand to include patients with lesser degree of heart failure who are receiving implantable cardioverter-defibrillators (ICDs) for standard indications. Other data support the use of CRT in patients with long-term dependence on ventricular pacing, in whom right ventricular single-site pacing may exacerbate left ventricular dysfunction and heart failure symptoms from pacing-induced electrical and mechanical dyssynchrony.
Work on enhancing CRT devices has been a focus of research innovation. Wireless remote monitoring of implantable devices in patients with heart failure may allow improved adherence and safety. In addition to monitoring lead and pulse generator parameters and stored arrhythmia events, some devices are capable of serial hemodynamic measurements, such as transthoracic impedance change. Improved leads and delivery systems, as well as increasing the experience of implanting physicians, permit coronary sinus leads to be placed with improved safety profile and success. An 86% success rate was achieved in biventricular pacemaker implantation on first attempt in the Cardiac Resynchronization–Heart Failure (CARE-HF) trial, while the rate improved to 92.5% in Multicenter Automatic Defibrillator Implantation Trial With Cardiac Resynchronization Therapy (MADIT-CRT) 4 years later. Further investigations to improve lead delivery include the use of an infrared illuminating system for coronary sinus cannulation.
Pacemaker indications
In August 2008, updated guidelines for device-based therapy of cardiac rhythm abnormalities were published by the American College of Cardiology, the American Heart Association, and the Heart Rhythm Society. Pacemaker indications for patients with sinus node dysfunction and AV block have remained consistent with previous guideline statements. Because of equivocal results in trials of pacing to prevent vasovagal syncope and reduce atrial fibrillation burden, pacemaker implantation for these indications has been deemphasized. The guidelines support CRT for medically optimized patients in New York Heart Association functional classes III and IV with left ventricular ejection fractions ≤35% and QRS durations ≥120 ms.
In October 2009, MADIT-CRT showed that CRT combined with ICDs lowered the risk for heart failure events in patients with New York Heart Association class I or II symptoms. The following month, Yu et al reported that biventricular pacing in patients with bradycardia and normal ejection fraction avoids adverse left ventricular remodeling and dysfunction seen with single-site pacing from the right ventricular apex. Although additional investigation may be required before these studies affect current guidelines, indications for CRT pacemakers could increase further. Rates of use of single- and dual-chamber pacemakers, in contrast, have stabilized.
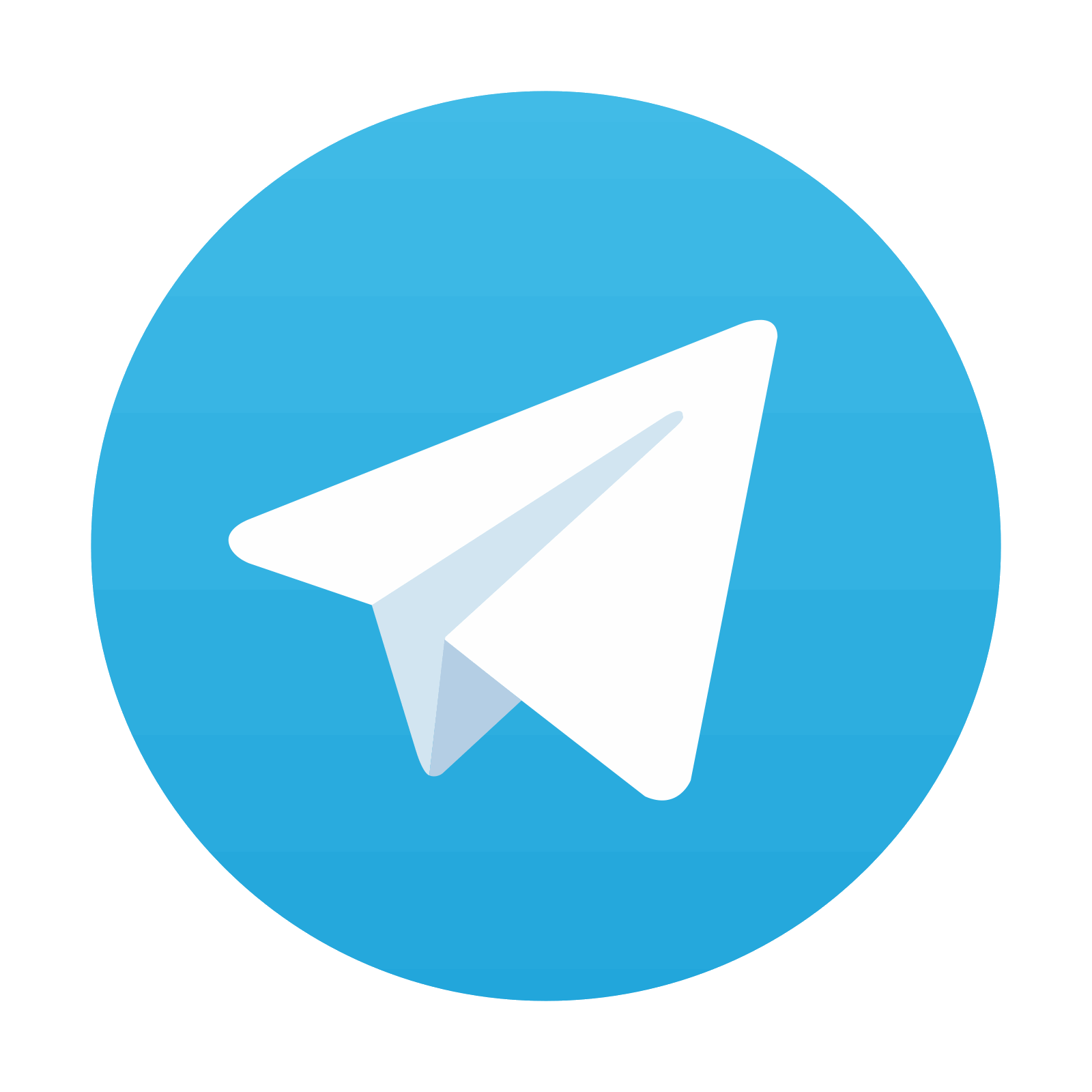
Stay updated, free articles. Join our Telegram channel
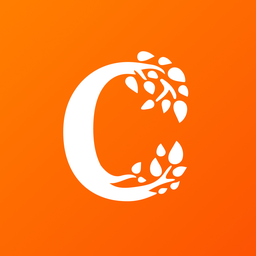
Full access? Get Clinical Tree
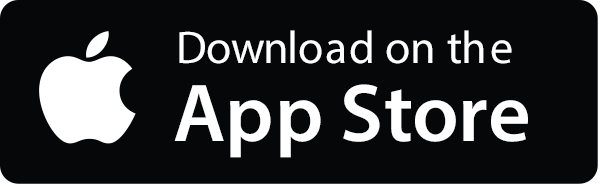
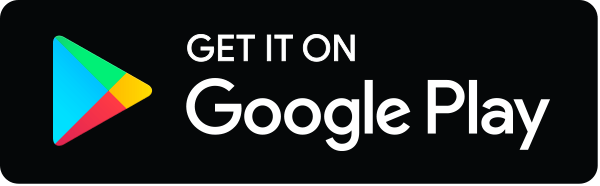