Tumor or organ
Motion and comments
Reference
Lung tumor
4D CT studies show that the majority of lung tumors move less than 1 cm in the cranio-caudal direction
[71]
Mediastinal lymph nodes
On 4D CT scans, nodes had a mean 3D motion of 0.68 cm (0.17–1.64 cm). No association was observed between 3D primary tumor and nodal motion
[94]
Pancreatic tumor / pancreas
On 4D CT scans the mean motion observed was 0.55 cm (
) in supero-inferior direction. Addition of a 1 cm margin accounted for all GTV motion in 97 % of patients

[35]
Liver tumor
On cine MRI mean liver tumor motion in patients without abdominal compression was 11.7 mm (range 4.8–23.3 mm) in the cranio-caudal direction
[25]
Kidney
4D CT studies have shown that motion is predominantly cranio-caudal and that there can be large inter-patient variation. Mean mobility in one study was 9.8 mm (2.5–30 mm) and 9 mm (2.5–20 mm) for the left and right kidney, respectively
[136]
Esophagus
In one 4D CT study, margins of 9 and 8 mm, respectively, were needed to account for all medio-lateral and dorso-ventral motion of the distal esophagus. The corresponding values for the proximal and mid-esophagus were 5/5 and 7/6 mm, respectively
[23]
A tenet of modern RT is that the target should be accurately identified, following which the high-dose treatment volume may be expanded into the surrounding tissues in order to create the clinical and planning target volumes (CTV and PTV). In Fig. 11.1 the different treatment-related volumes recommended by the International Commission on Radiation Units and Measurements (ICRU) are illustrated. Although the accurate identification of the gross tumor volume (GTV) is essential, it remains a weak link in the radiotherapy treatment planning (RTP) process. Defining the GTV is often difficult due to the lack of a sharply demarcated tumor boundary and it is subject to inter-observer variation (Fig. 11.2). Tumor motion creates additional challenges, and an important component of 4D RTP for motion-affected tumors is the definition of an appropriate internal target volume (ITV). The ITV is then typically expanded to create the planning target volume (PTV) which accounts for all uncertainties in the treatment delivery process.
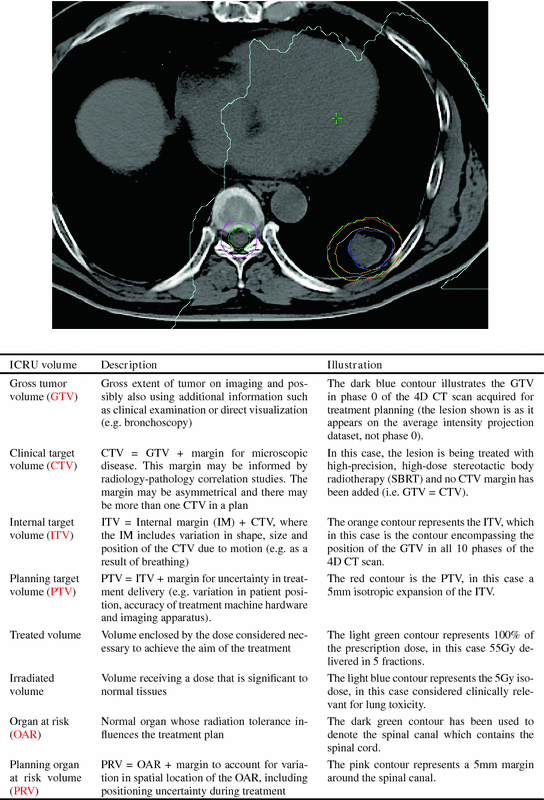
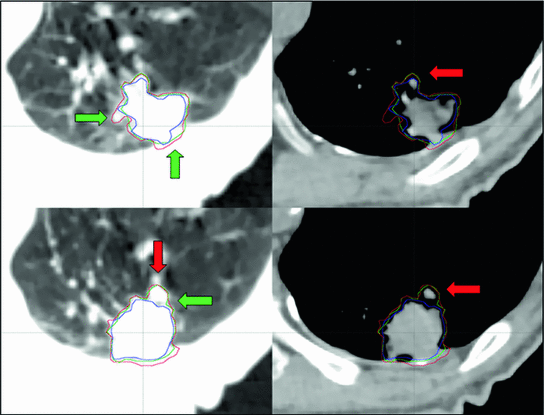
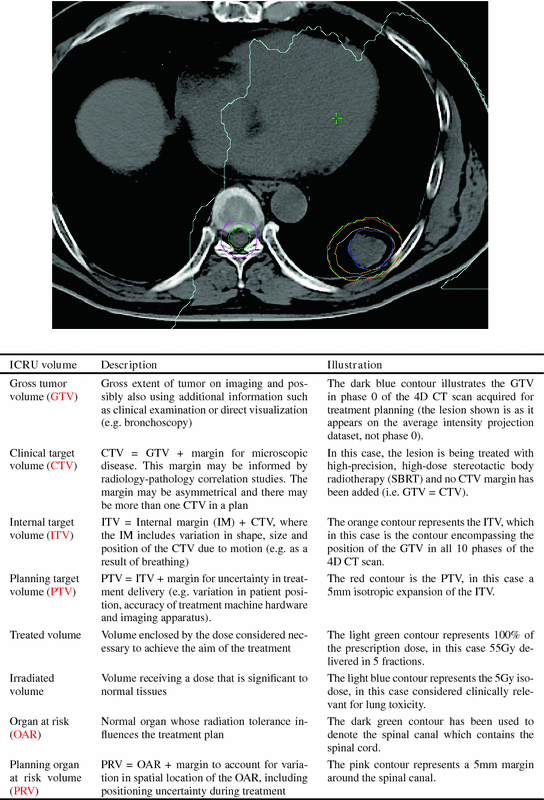
Fig. 11.1
Illustration and description of treatment volumes and concepts described in ICRU Report 62 [48]
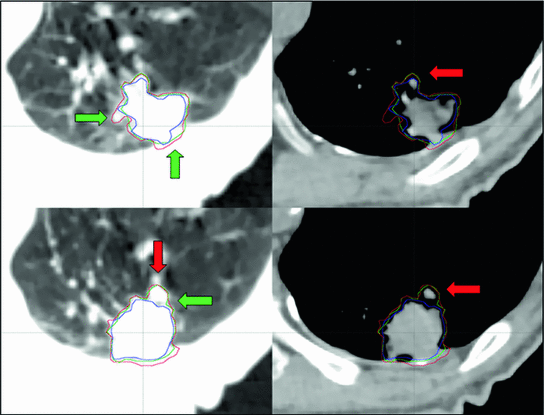
Fig. 11.2
An example of contouring variations by 3 clinicians who delineated a peripheral lung tumor on a single phase from a 4D CT (red, green and blue contours) using information from lung (left panel) and mediastinal (right panel) window/level settings. Illustrative differences in interpretation of anatomical data are highlighted (green arrows). Blood vessels (red arrow) are among the normal structures that can contribute to such inter-clinician variation
In this paradigm, the more uncertainty there is, the more expansion will be necessary, with a corresponding increase in risk of damage to surrounding normal tissues and decrease in the therapeutic ratio, where the therapeutic ratio is defined as:
It is therefore necessary to control and where possible reduce uncertainty in target designation and treatment delivery at the level of the individual patient.

Historically, RTP in the thoracic and abdominal regions has used 2- or 3-dimensional images acquired under uncoached free-breathing conditions, irrespective of whether the target tumor is moving or not. Once the GTV has been delineated, standard margins are commonly added in order to account for motion. However, the use of such generic margins does not usually take into account the location of the target in its motion trajectory, and furthermore might under, or over-estimate motion in individual patients with subsequent risks of missing the target during treatment, or irradiating excessive normal tissue [4]. RTP based on the use of conventional ‘fast’ CT images acquired at a random phase of the respiratory cycle therefore risks introducing systematic errors into treatment planning and delivery. In lung cancer, for example, such systematic errors may remain undetected if set-up at the treatment unit does not include daily [44] or frequent visualization of the tumor, or a robust surrogate/fidicual marker, and could occur if image-guidance is based solely on orthogonal kilovoltage images of the bony anatomy [103]. This increases the risk that the calculated tumor doses will not correspond to the delivered dose which may impact on both TCP and NTCP. The recent availability of on-line soft-tissue verification of tumor position can reduce the risk of systematic errors arising from a non-representative RTP scan and allow for modification/adaptation in treatment planning margins. Such strategies also require that attention is paid to the location of critical normal structures.
4-dimensional (4D) imaging permits patient-specific treatment planning by incorporating temporal information about tumor and organ at risk (OAR) motion, thereby allowing for the use of individualized margins. A general approach for integrating 4D image information into the treatment planning workflow can be divided into the following steps:
These steps are discussed in the next sections and strategies to integrate tumor and organ motion are reviewed. Strategies for respiratory motion management during the radiotherapy treatment are reviewed in Sect. 11.6 and methods for imaging-based on-line verification are discussed in Sect. 11.7. The rapid developments in image acquisition and IGRT over recent years means that 4D CT is now widely considered to be the standard for treatment planning in the chest and upper abdomen, and that on-line volumetric positional verification with cone-beam CT (CBCT) is increasingly used for image-guided treatment delivery. Both 4D CT and CBCT can be readily integrated into clinical workflows.
(i)
acquisition of 4D images for radiotherapy planning,
(ii)
target and OAR delineation with appropriate use of motion information from 4D imaging data and addition of appropriate margins,
(iii)
generating a treatment plan with a dose distribution that adequately treats the volume encapsulating the observed tumor motion, and
(iv)
quality assurance to verify that the treatment plan can be robustly delivered.
11.2 4D Imaging for Radiotherapy Planning
Individual approaches to 4D imaging are briefly reviewed, and clinical examples will largely focus on lung cancer, in particular early-stage non-small-cell lung cancer (NSCLC), where considerable work has been performed on 4D imaging. The most common contemporary imaging technique for identifying tumor motion is 4D computed tomography (4D CT). In other approaches, additional spatio-temporal images are acquired beside the 3D planning CT to identify the extent of tumor and organ motion. Usually, in these approaches a co-registration of the additional images with the planning CT is necessary either as an integral part of the image acquisition technique (e.g. PET/CT) or by using registration algorithms as described in Chaps. 5 and 6. Note that depending on the 4D imaging strategy used, and the extent of image acquisition, the final set of treatment planning images may or may not include 4D information about organs at risk in addition to the target. In some scenarios this will be an important clinical consideration and if there is no 4D information about OAR motion, then alternative strategies (such as the application of an appropriate PRV margin) may be necessary in order to design an acceptable treatment plan.
11.2.1 Multiple Conventional CT Scans
Acquiring multiple fast CT scans at random during the same session allows for a better assessment of breathing motion than a single scan. There are several possible approaches to this. In breath-hold end tidal CT, 3 fast scans may be acquired, one in free breathing and one each during quiet end inspiratory and expiratory breath-hold [118]. Alternative techniques involve the acquisition of multiple scans at random points in the breathing cycle while the patient is in the treatment position. The initial scan typically includes all of the required cranio-caudal volume for treatment planning purposes, whilst subsequent scans are limited to the region of the tumor. All the scans are co-registered to each other, and the GTV can then be contoured on each scan separately. These contours can be combined on the full-length scan, which is used for treatment planning, to generate an internal target volume (ITV). Underberg et al. compared the ITV from a single respiration-correlated 4D CT scan (
) to one derived from 6 conventional CT scans (
) in treatment planning for stereotactic lung RT [128]. This study found that the
was comparable to, or larger than the
, suggesting that the former more reliably identified the true extent of tumor motion. The same study also evaluated expansion of the GTV derived from a standard CT scan by an isotropic margin of 10 mm. It found that although this lead to a PTV that was on average twice the size of that formed by a 3 mm expansion of the
and
there was nonetheless incomplete geometric coverage of the two tumors showing most mobility. This study highlights the potential benefits of systematically accounting for tumor motion, reducing in some cases both the risk of tumor miss and the volume of normal tissue in the PTV.






11.2.2 Fluoroscopy
Fluoroscopy can allow selected tumors to be imaged in more than one dimension and the data to be used in several different ways. Clinical examples include (1) combining digital fluoroscopy loops with digitally-reconstructed radiographs from a conventional CT scan in order to derive a patient-specific ITV [119], (2) following CT-based RTP using generic treatment planning margins, fluoroscopy can be performed using a conventional simulator in order to verify that the planning margins adequately encompass tumor motion, (3) use of on-line/on-board kilo- or mega-voltage (kV or MV) fluoroscopic imaging to verify tumor motion and the adequacy of the PTV and dosimetric tumor coverage immediately prior to daily treatments. All the above can be performed with or without implanted fiducial markers. Although they may be easier to identify on fluoroscopy than the tumor itself, fiducial markers are not widely used in patient care as they introduce additional risks for the patient and may present logistical and technical challenges [116]. In practice therefore, while selected peripheral lung tumors may be visualised with fiducial-free fluoroscopy, this is less often the case for moving tumors located more centrally in the thorax (which even if seen, may only be partially visible), and for lesions within the abdomen. This has resulted in guidelines that have cautioned against using fluoroscopy alone as a primary method with which to characterize lung tumor motion for RTP [114].
11.2.3 Slow CT
This technique differs from conventional fast CT in that the period of scanner rotation is slowed down, for example to 4 s, which is often long enough to capture respiratory tumor motion during a full respiratory cycle. This results in a blurred image that can be registered to a conventional CT for RT planning. Lagerwaard et al. compared conventional CT and slow CT scans of peripheral lung tumors and reported that the latter better accounted for motion, generating larger and more reproducible target volumes [60]. A subsequent study evaluated the number of slow CT scans required for treatment planning by comparison with a reference target volume derived from a total of 3 fast and 3 slow CT scans [22]. This found that a single slow CT scan with a 5 mm isotropic expansion was sufficient to cover 99 % of the reference volume. Phantom studies have shown that modern CT scanners with more limited ability to slow down the gantry rotation time (e.g. to 1.5 s) can still be used to capture information about target motion [18]. A drawback of slow CT is the fact that central tumors, and lesions adjacent to the mediastinum and diaphragm may be poorly visualized using this approach.
11.2.4 4-Dimensional CT (4D CT)
While combinations of conventional CT scans can be used to approximate tumor motion, they may be susceptible to motion artifact. Several authors have now described methods for generating 4D CT images that oversample the same anatomical location during respiration and then ‘sort’ or ‘bin’ images that have been allocated to the appropriate respiratory phase based, for example, on matching either the internal anatomy or an external respiratory signal (see [92, 109, 137] and Chaps. 2 and 3). 4D CT has now become the preferred modality for imaging tumor motion in routine clinical practice. This approach allows for discrete image sets to be generated for individual respiratory phases, as well as average and maximum intensity projection datasets (AIP, MIP respectively). However, 4D CT images are prone to imaging artifacts (see Chap. 2). These artifacts may arise due to higher motion velocity [84], greater motion amplitude or variations in breathing patterns [61, 97, 112], incorrect binning of images and missing images [1]. Such artifacts can affect the image quality and fidelity of the AIP and MIP and may affect the target delineation [12, 13]. A greater variability in CT number (Hounsfield units) than is seen with conventional CT has also been reported [123]. In 50 patients undergoing 4D CT for thoracic or abdominal radiotherapy, Yamamoto et al. reported artifacts in 90 % in the region of diaphragm or heart (4.4–56 mm in size), and 6 of 20 patients exhibited at least one artifact in a lung or mediastinal tumor. They concluded that significant improvement was still needed in 4D CT imaging [147].
It is important, therefore, that 4D CT workflows should include an evaluation of the respiratory signal at the time of imaging and also the quality of the 4D CT images (see Fig. 11.3). This should ideally be performed prior to the patient coming off the CT couch and leaving the facility, so that they can be rescanned if excessive artifacts are observed.
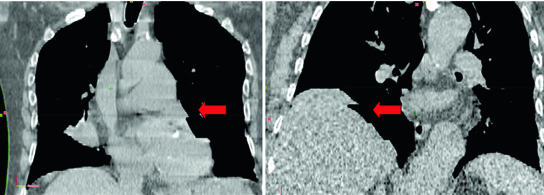
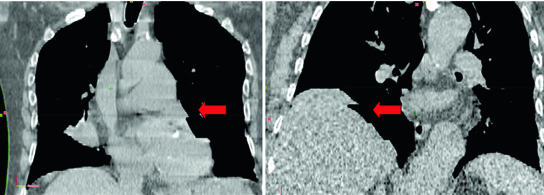
Fig. 11.3
4D CT images of the thorax acquired under uncoached, free-breathing conditions using a 16-slice CT scan and a cine CT technique. Typical artifacts (arrows) are seen in the region of the mediastinum (left) and diaphragm (right)
Strategies to reduce the variability in breathing pattern include respiratory coaching. Combined audio-visual (AV) signals are one way of trying to regularize breathing however not all patients may be able to comply with this. Indeed Neicu et al. found that approximately half of the patients studied could not simultaneously follow audio and visual prompts [86]. For those patients that are coached, differences in breathing amplitude can be expected between coached and free-breathing conditions [98] indicating that if it is used for 4D CT planning then coaching should also be used during treatment. In an early study on AV coaching, Kini et al. found that audio coaching tended to improve the breathing frequency and visual coaching the amplitude, although each was somewhat at the expense of the other [54]. Audio coaching has also been shown to improve the correlation, reproducibility and phase shift between lung tumor motion and abdominal marker displacement, again perhaps at the expense of an increase in the depth of breathing and absolute tumor motion [82]. Differences in ITV position exceeding 5 mm between coached and uncoached 4D CT scans have been detected in up to 56 % of mobile lung tumors, with both end-inspiration and end-expiration positions susceptible to displacements [40]. In practice, the requirement for consistent coaching between 4D CT and treatment delivery [40], time, complexity and equipment has probably reduced the widespread uptake of this technique.
11.2.5 Positron Emission Tomography (PET)
PET imaging often involves the acquisition of data over several minutes in the same position, which means that a moving tumor will produce a blurred image. It has been postulated that PET images may define the motion envelope of the tumor. Identifying this envelope with a high degree of certainty and spatial confidence is an ongoing challenge in PET imaging. An early paper on this topic described a phantom study of moving spheres imaged with conventional CT and PET [14]. Uniform PTV margins added were 7.5 mm to the PET-based target and 7.5, 10 and 15 mm to the CT-based target. The CT images were prone to distortion and underestimated the motion envelope while the PET targets reflected the shape of the spheres and overestimated the true envelope. The 7.5 mm PET margin was reported to be adequate to avoid a geographic miss, whereas the CT-target required 15 mm margin that would have translated into more normal tissue being treated in-vivo. A key drawback of PET is that it suffers from limited spatial resolution and there is persisting uncertainty in how to segment the motion envelope of the tumor. Not surprisingly therefore, a great variety of approaches have been described for PET-based contouring [88, 144]. The challenges of knowing what represents the ground truth and in establishing appropriate segmentation parameters for mobile and non-mobile tumors, has led to an increasing interest in radiology-pathology correlation studies for PET and other imaging modalities [21, 77]. Many tumors have dominant motion in one direction (e.g. cranio-caudal) and it has been demonstrated that the size of the target, extent of motion, velocity and background activity may all affect PET images [107]. Despite this, most PET segmentation algorithms use isotropic segmentation methods to segment moving targets on 3D PET images, which it has been demonstrated can ‘lose’ PET data when the target is moving [27, 70]. We feel that anisotropic segmentation merits further evaluation as a means of detecting motion envelopes.
Key limitations of PET imaging must be acknowledged in order to ensure appropriate clinical use of this modality. The ability of PET to be used to guide biological sub-volume boosting or dose-painting has been studied by Perrin et al. who felt that with the available imaging quality ‘simple’ techniques seemed possible at intermediate contrast levels, which they defined as a tumor-background ratio of about 10 [96]. The advent of 4D PET [85] with attenuation maps derived from 4D CT has made it possible to recover some of the ‘lost’ metabolic information from a moving tumor. However, several issues remain unresolved such as the optimum number of phases to use, which represents a balance between reducing the amount of motion in any given phase by increasing the number of phases, at the expense of an increased signal to noise ratio [8, 85, 95]. The optimum integration of 3 and 4D PET-CT imaging into radiation treatment planning is still uncertain, and the specific role of metabolic imaging is likely to vary between tumor sites and whether they are mobile or not. At the present time, PET cannot be recommended as a primary modality for the routine identification of tumor motion in RTP.
11.2.6 4D Cine Magnetic Resonance Imaging (MRI)
Cine MRI has been used by a number of authors to image tumor motion at anatomical sites including pancreas, liver, bladder, cervix and prostate [16, 33, 75]. 4D 1.5 Tesla (1.5 T) fast low angle shot (FLASH) MRI has also been used to characterize complex breathing motion in patients with diaphragmatic paralysis due to lung cancer [24]. A recent investigation studied the ability of 4D CT, 4D MRI and 4D CBCT to detect nodule size and displacement in a dynamic ex-vivo lung model [9]. All modalities were found to have underestimated lesion displacement, and both 4D MRI and 4D CBCT overestimated lesion size. Kirilova et al. used T2-weighted single shot fast spin echo MRI sequences to image liver tumor motion in 3 dimensions, but this approach did not correlate well with fluoroscopic images of cranio-caudal diaphragmatic motion [55]. This, together with other studies, highlights the challenges of integrating multi-modal imaging studies acquired at different time points under different conditions, and in determining tumor motion with high levels of certainty. In terms of the relationship of tumor motion to anatomical surrogates, 3T cine MRI imaging of the pancreas has shown that the position of the tumor correlates poorly with that of the diaphragm (and abdominal wall), which can lead to inadequacies with gating based on these structures [30]. This study also demonstrated that tumor motion was greater than expected, requiring margins of 20, 7, 10 and 4 mm in inferior, superior, anterior and posterior directions to achieve 99 % geometric target coverage. Cine fast gradient echo MRI has also been used to show that lung blood vessels (used an internal surrogate for lung structures) have a variable correlation with different skin surface sites. The strength of correlation was influenced by the locations of the internal and external surrogates and the breathing pattern [57].
11.2.7 Comparison of 4D Imaging Methods
Multiple methods of imaging tumor motion have been described prompting several questions including (1) are there cautionary notes with any of these imaging modalities and (2) is one of them superior to the others?
When tumor motion in all directions on 4D CT in 29 patients was compared with motion assessed in antero-posterior projection using fluoroscopy, the latter method resulted in a mean ITV that was 52 % larger, with 4D CT generating a larger ITV in only 3 patients [134]. This study again demonstrated some of limitations of fluoroscopy, which did not allow motion to be evaluated in at least one direction in 8 of 29 patients. Underberg et al. found that a single 4D CT produced a PTV (ITV + 3 mm) that was comparable to or larger than that derived from a combination of 6 conventional fast CT scans (again with a 3 mm PTV margin) in patients with stage I NSCLC, indicating that the 4D CT more completely accounted for tumor motion [128]. This study highlighted the potential of 4D CT to simultaneously account for tumor motion and, in some patients, also reduce normal tissue irradiation. Nakamura et al. have looked at whether slow CT captures 4D CT motion, finding that even when tumor motion is less than 8 mm, it may not be able to do so [83]. In situations where no 4D CT is available a combination of end-tidal breath-hold images may best describe tumor motion [118]. In relation to this, Hunjan et al. have evaluated the accuracy of end-tidal image acquisition when guided by the location of an external abdominal marker and concluded that relying solely on an external abdominal marker as a surrogate for the extremes of free breathing may be subject to error [47]. The same group have recently reported that the absolute difference between mean free breathing and breath hold marker displacement did not correlate well with the absolute difference between GTV centroid positions on free breathing 4D CT and breath-hold images, again highlighting the potential limitations of using external surrogates of tumor motion [46]. Although they represent an improvement over conventional fast CT and generic margins, some uncertainty still needs to be assumed when imaging tumor motion with current methods including 4D CT.
There are currently no clinical outcome data to indicate the superiority of one method of imaging motion over another. Lung stereotactic body radiotherapy often involves treating relatively small tumors in patients who are unfit to undergo surgery because of medical morbidity including chronic obstructive pulmonary disease (COPD) [59]. High radiation doses are used (e.g. 3 fractions of 18 Gy or 5 fractions of 11 Gy), which indicates a need for precise and accurate treatment in order to limit the dose to organs at risk (OAR) and reduce the likelihood of geographic miss. The information from selected publications on the treatment planning methods and motion management strategy can be contrasted with reported clinical outcomes (see Table 11.2). In summary, local control rates in excess of 90 % have been reported after stereotactic lung RT for relatively small, early-stage lung tumors, and control rates are comparable across a variety of treatment planning and delivery methods, including those that use generic planning margins and image-guidance based on surrogate anatomy. This therefore makes it difficult to currently ascribe superiority to one technical approach over another on the basis of clinical outcome. Possible responses to this could include (1) differences in the rates of local recurrences were masked by the high rates of non-cancer mortality due to extensive co-morbidity in treated patients, (2) the studies were not designed to detect gains from using a specific technology, or (3) the use of very high doses may have been forgiving of any imperfection in accounting for motion or inaccuracy in treatment delivery, which could mask the benefit from individual technologies.This final point contrasts with some reports suggesting that at lower biological doses, the transition from 2D RT to 3D conformal RT (CRT), while using similar conventional dose-fractionation schedules for stage I non-small cell lung cancer (NSCLC), and from 3D CRT to 4D CT/intensity modulated RT (IMRT) in locally advanced NSCLC, may be associated with improvements in clinical outcome, including patient survival [29, 66].
Table 11.2
Treatment planning, image-guidance strategies and local control in selected stereotactic lung radiotherapy series’
Reference | Treatment planning and margins | Image-guidance strategy | Patients (n) and follow up (FU) | Local control (LC) |
---|---|---|---|---|
Grills et al. JCO, 2010 (abstract) [36] | Not specified | On-line CBCT | ![]() | Kaplan Meier estimated 92 % LC at 2 years |
Timmerman et al. JAMA 2010 [127] | CT plus fluoroscopy, or 4D CT, no CTV expansion maximum 5 mm axial and 10 mm cranio-caudal PTV margins, motion control if it exceeds these margins | ‘Image-guidance capable of confirming the position of the target’ | ![]() | Kaplan Meier estimated 97.6 % LC at 3 years |
Baumann et al. JCO, 2009 [5] | CT, 1–2 mm GTV-CTV margin, 5–10 mm transversal and 10 mm longitudinal CTV-PTV margins | CT before treatment to verify reproducibility of target within the stereotactic system | ![]() | Kaplan Meier estimated 92 % LC at 3 years |
Lagerwaard et al. IJROBP, 2008 [59] | 4D CT derived ITV with 3 m ITV-PTV margin | Stereoscopic x-rays with match on spine | ![]() | Kaplan Meier estimated 93 % LC at 2 years |
In contrast, review of non-clinical end-points suggest an apparent advantage to specific technologies frequently used in lung SBRT, such as the use of tumor-based matching for on-line volumetric guidance, or treatment planning with heterogeneity correction [121, 146]. Determining the contribution of technological advances, such as optimal motion management, to clinical outcomes can therefore be difficult. One view is that the paradigm shift in radiation treatment is primarily radiobiological or related to improvements in combined modality therapies, and that one of the real (although perhaps less tangible) benefits of technological advances has been to enhance the confidence of the clinical team in delivering such treatments and increase their uptake into mainstream clinical practice as well as helping to extend the application of effective dose-fractionation schedules to ever more challenging situations (e.g. where the tumor is in closer proximity to critical structures) [34].
11.3 Target Delineation in 4D RTP
In this section, we discuss target delineation (GTV and ITV) in 4D RTP based on 4D CT images. In many of the the following examples relating to studies carried out for the treatment of early stage lung cancer, no CTV margin is used, and so the ITV contour represents the GTV plus an internal margin.
A number of approaches are in use for contouring the target volume [120], including
Note that these methods cannot all be considered equal. For example, it is important to note that the extremes of motion may not be represented by extremes of the breathing cycle, and that the maxima of cranio-caudal, medio-lateral and antero-posterior motion may be located in different phases. Evaluating early stage lung tumors in different 4D CT phases, Wu et al. concluded that the major effect of respiration on lung tumors was translational, as the addition of rotation or deformation had little impact on registration metrics such as the overlap index [143].
(1)
contouring a GTV in all 10 phases, and then combining contours of all phases to define the ITV,
(2)
contouring of only end-inspiration and end-expiration images in order to derive a motion encompassing volume,
(3)
presenting the 4D CT images as a maximum intensity projections image (MIP), reflecting the maximum data value for a given pixel in all phases of the 4D CT and then contouring the tumor edge as it appears on the MIP, and
(4)
a modified MIP approach that involves modifying the ITV contour derived from the tumor edge as it appears on the MIP images, with information from all 4D CT phases, or selected phases that represent extremes of motion.
The increased anatomical detail provided by 4D CT imaging also allows the mobility of mediastinal lymph nodes and organs at risk such as the esophagus and kidneys to be identified and where necessary taken into account during treatment planning [23, 94, 136]. Although the use of a planning organ at risk volume (PRV) was conceptualized in ICRU report 62 to incorporate uncertainties in OAR localization and shape, it is currently less clear how best to evaluate and trade-off dose-volume histograms predicated on constructs such as the PRV and OAR volumes defined using 4D CT imaging, for example if the time spent by an OAR at the extreme of motion is very short, or there is uncertainty over the consequences of partial organ irradiation [104].
Can MIP images adequately reproduce the ITV from all 10 phases of the breathing cycle? This issue was addressed using 4D CT images from 12 patients with stage I lung cancer and a phantom study which reported a ratio between 10-phase GTV and MIP of 1.04 [130]. The former study was in early-stage NSCLC and suggested that the MIP was a quick and fairly reliable way to identify tumor motion. For more advanced tumors and in patients with irregular breathing, however, the MIP may be less reliable and risks underestimating the ITV defined by using all 10-phases [80]. In practice, the MIP may be viewed as a way of approximating the motion envelope that can subsequently be refined by incorporating additional information from individual respiratory phases. This is consistent with the findings of Rietzel et al. [108] and Ezhil et al. [28]. The latter found that in 27 patients with stage I or III NSCLC the ITV derived from the MIP and modified by using all 10 phases of the 4D CT agreed most closely with the ITV defined from all 10 respiratory phases, which was underestimated by using the MIP or the 0 and 50 % respiratory phases alone. The MIP may be unreliable for example where the tumor is close to chest wall, blood vessels, diaphragm or mediastinal structures (denser, mobile structures). Although an ITV derived from MIP-modified identifies an approximate motion envelope, it does not help to identify microscopic disease, which means that in some instances an additional margin for the CTV may be considered desirable. In addition there is no guarantee that the motion of the tumor during treatment will be the same as it was when the planning 4D CT was acquired.
Inter-observer variations and reproducibility of target volume delineation are key factors contributing to overall quality of treatment [115], and this remains the case in the 4D CT era [72]. This provides an impetus to the development of automated contouring for target volume delineation. The use of deformable registration as introduced in Chaps. 5–7 to auto-propagate a single GTV may reduce inter-clinician variations in generating ITV’s for peripheral lung tumors [132]. Automated contouring or registration-based contour propagation is impeded by the aforementioned possible imaging artifacts in 4D CT datasets. Ehler et al. presented a system to auto-segment the GTV using 4D CT with up to 15 % phase binning errors [26]. Further, challenges for auto-segmentation include identifying structures when they have inherently indistinct boundaries or the imaging modality used for treatment planning has low resolving power for the structure in question and cannot differentiate it well from surrounding tissues. This means that such contours still need to be verified by the clinician (see Fig. 11.4).
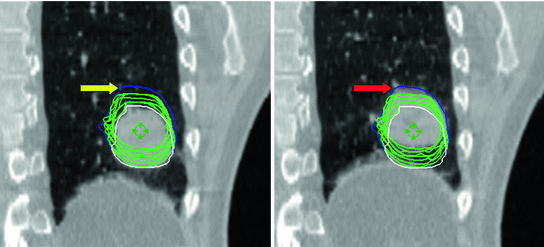
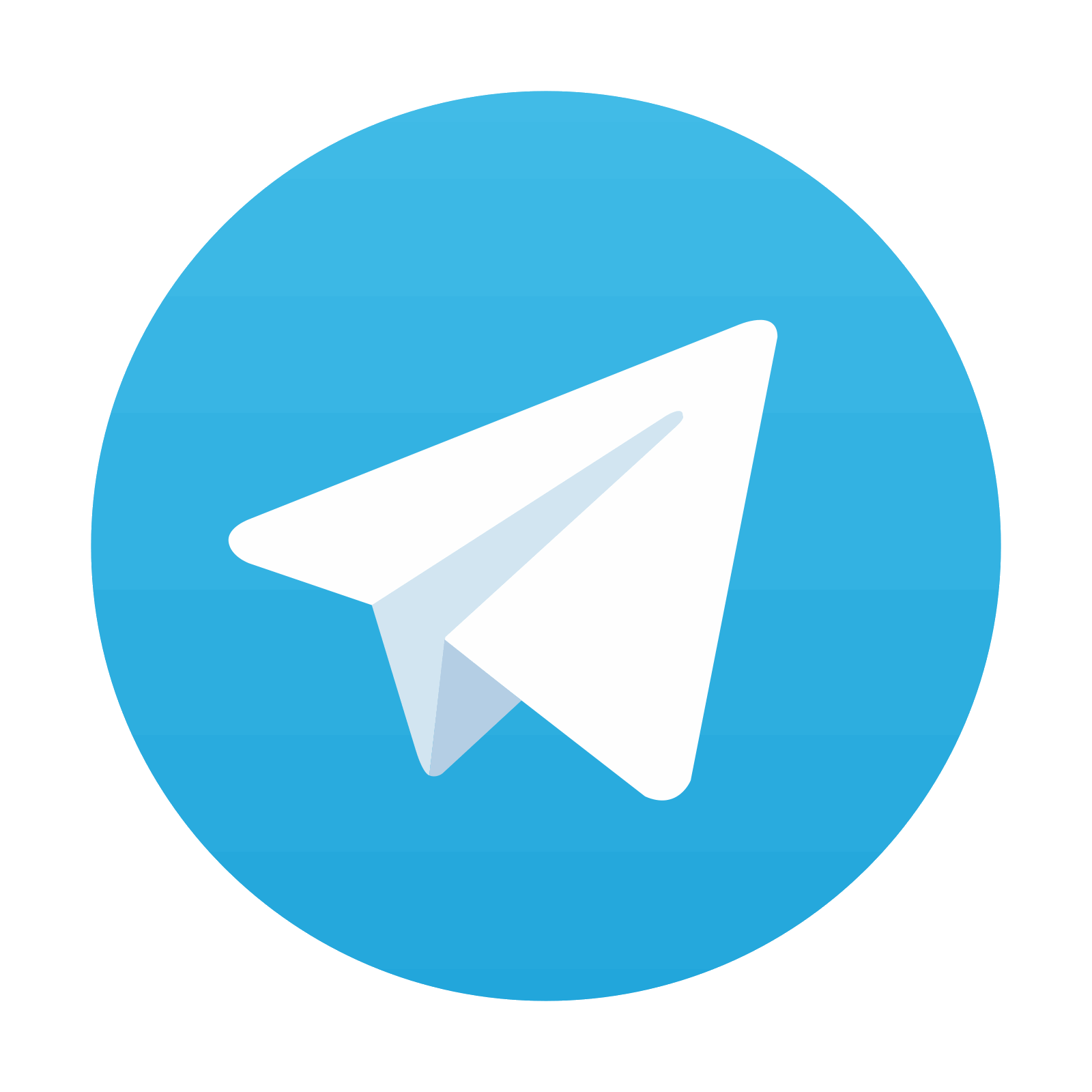
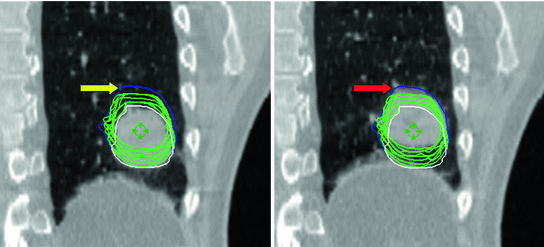
Fig. 11.4
Example showing the use of deformable registration for tumor segmentation. A single manual GTV contour at end inspiration (white contour) was successfully deformed to each of the other phases of 4D CT (green contours). The blue contour encompasses the manual contours of a single observer who has delineated the GTV on all 10 phases of the same 4D CT. Differences between the green and blue contours (yellow arrow) are seen on the end-inspiration data set (left panel) at the cranial extent of contours propagated using deformable registration and the manually derived contour. Inspection of the end-exhale image of 4D CT (right panel) shows a region of increased CT density (red arrow) which was incorporated by the clinician, but not by the software-based registration. This highlights the need for clinicians to verify all automated contours
< div class='tao-gold-member'>
Only gold members can continue reading. Log In or Register a > to continue
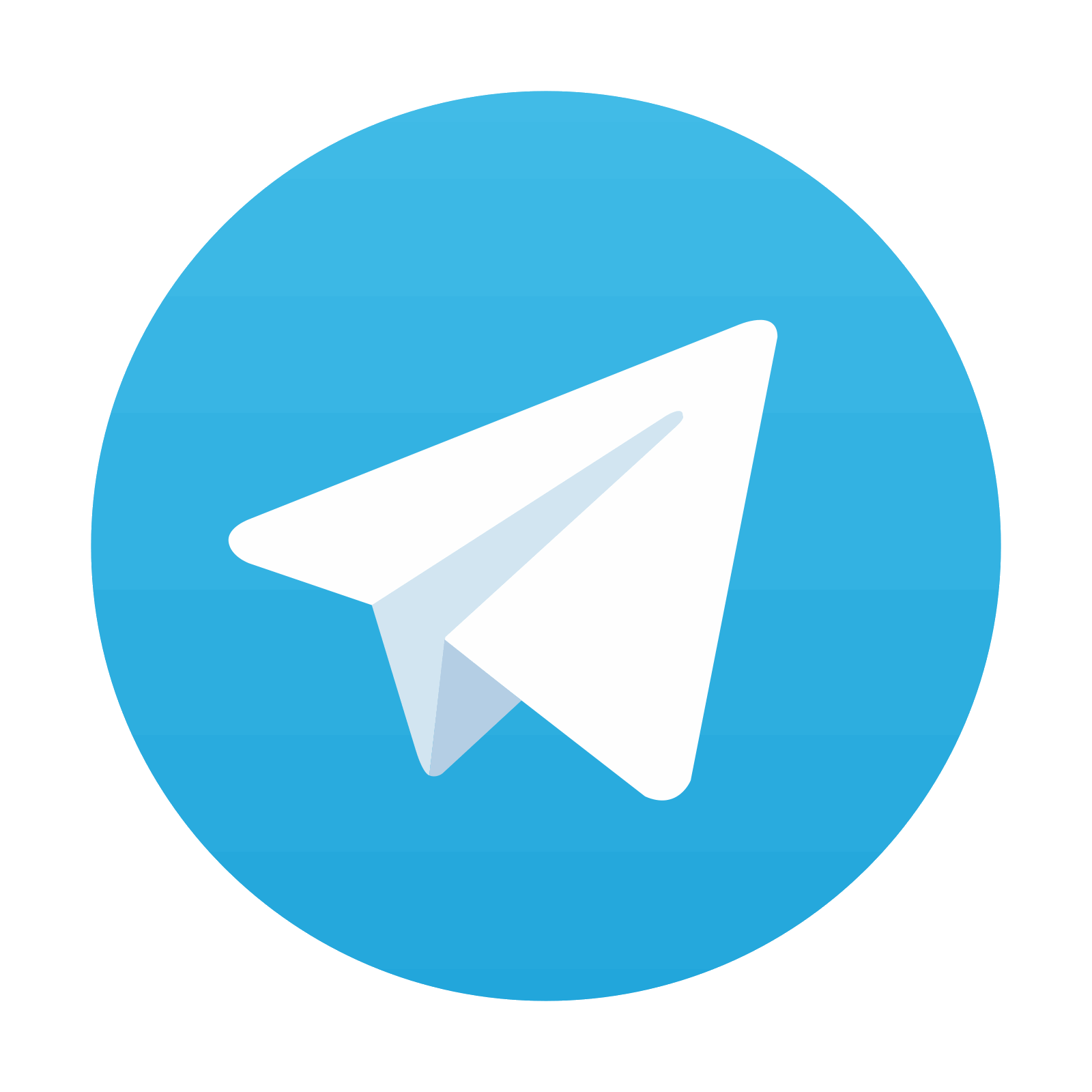
Stay updated, free articles. Join our Telegram channel
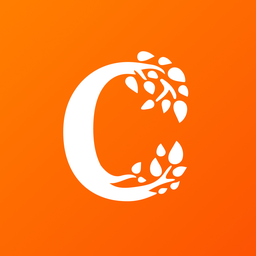
Full access? Get Clinical Tree
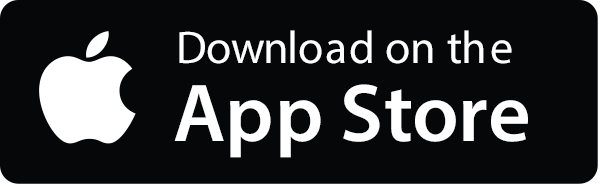
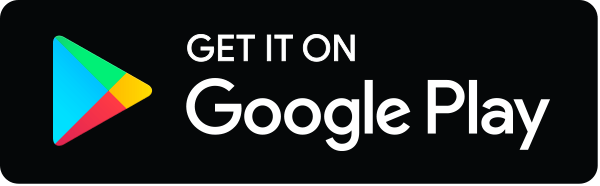
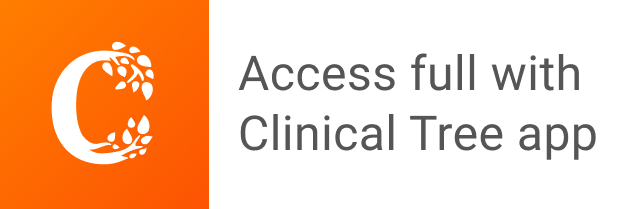