Our genetic background and way of life as well as environmental factors are closely related and synergistically control our physiology and health status. Though we are the imprint of our genes, a plethora of scientific evidence confirms that lifestyle stimuli such as diet, physical activity, stress, smoking, alcohol consumption, and pollutants, among others, can influence the genome by triggering epigenetic mechanisms that potentially can cause changes in gene expression (Alegría‐Torres, Baccarelli, & Bollati, 2011; Ramsay, 2015). Epigenetic alterations occur during the entire lifespan, from conception to adulthood. In the broad sense of the term, epigenetics, which literally means “above the genes”, are the bridge between genotype and phenotype and modify genome function by offering a way to control the expression of genes (Dupont, Armant, & Brenner, 2009). Admittedly, internal and external cues influence the epigenetic landscape, disrupt genetic programming, and eventually modify DNA, mainly through regulating which genes are turned on or off (Peral‐Sanchez, Hojeij, Ojeda, Steegers‐Theunissen, & Willaime‐Morawek, 2021). The term “epigenetics” was first introduced by Conrad Waddington in 1942 (Waddington, 1956) who referred to the study of epigenesis, namely how phenotypes derive from genotypes during development (Casadesús & Noyer‐Weidner, 2013; Dupont et al., 2009). He defined epigenetics as “the branch of biology that studies the causal interactions between genes and their products which bring the phenotype into being” (Noble, 2015; Waddington, 1956). With the rapid growth of genetics, the meaning of the term has undergone several transitions and is now used to describe the study of changes in gene function that are heritable and are not attributed to alterations in the DNA sequence (Deans & Maggert, 2015). While the sequence of DNA remains constant, the epigenetic state of the genome (i.e., epigenome) changes dynamically in the cells of different tissues and, by using many coordinating enzymes, regulates the expression of genes to alter the phenotypes in different cell types (Lacal & Ventura, 2018). Epigenetic mechanisms can influence genes at the transcriptional and post‐transcriptional levels and/or at the translational and post‐translational level (Gibney & Nolan, 2010). FIGURE 2.1 Transgenerational epigenetic inheritance. Source: (Lacal & Ventura, 2018 / Frontiers Media S.A / CC BY‐4.0). Epigenetic and genetic information are both inherited, but contrary to genetic information, epigenetic data are reversible (called epigenetic plasticity) and may be affected by various stimuli or environmental factors (Bohacek & Mansuy, 2013). These changes may persist through multiple cell divisions, even for all of a cell’s life, and may also be transferred to the offspring, lasting for multiple generations via trans‐generational epigenetic inheritance (Wei, Huang, Yang, & Kang, 2017). The knowledge of the mechanism by which epigenetic information is transmitted is poor, but it is known to be transferred differently between sexes. More specifically, an exposure to an environmental factor, such as a toxicant, to a gestating F0 female individual promotes an epigenetic alteration in the germ cell programming of the F1 generation fetus (Figure 2.1). The altered germ cell epigenetics is then transmitted in the second (2) and the third (3) generations (Lacal & Ventura, 2018; Nilsson, Ben Maamar & Skinner, 2022), and consequently, only the fourth generation can be considered as “event free”. An exposure to an environmental factor in the father, it can only modify his sperm, producing an epigenetic change in the F1 generation, effecting a reliable non‐genetic inheritance in the third generation (Donkin & Barrès, 2018; Lacal & Ventura, 2018). The fundamental molecular mechanisms that mediate epigenetic modulation are DNA methylation, histone posttranslational modification, and non‐coding RNA regulation (Dupont et al., 2009) (Figure 2.2). The above‐mentioned epigenetic mechanisms are controlled by several enzymes, the writers that add chemical residues to DNA and histones, the erasers that remove them, and the readers, specialized domain‐containing proteins that identify and interpret those modifications (Biswas & Rao, 2018). The most‐studied epigenetic mechanism is DNA methylation, which includes the addition of a methyl group (CH3) at the nucleotide cytosine (Dupont et al., 2009). The most common DNA methylation process is the covalent addition of the methyl group at the 5‐carbon of the cytosine ring resulting in 5‐methylcytosine (5‐mC). In mammals, DNA methylation occurs at cytosines in any context of the genome; in somatic cells methylation occurs almost exclusively in a cytosine–guanine site (CpGs), while in embryonic stem cells (ESCs) as much as a quarter of all methylation appears in a non‐CpG context. When methyl groups are present on a gene, that gene is turned off or silenced, and a protein is not produced from that gene. Furthermore, histone modifications are key epigenetic regulators that control chromatin structure and gene transcription, thereby affecting various cellular phenotypes (Molina‐Serrano, Kyriakou, & Kirmizis, 2019). Histone modification includes multiple, mostly reversible, chemical alterations (e.g., acetylation, methylation, and phosphorylation) of nucleosome core histones, particularly at their N‐terminal sequences, and of the linker histone H1. Due to this characteristic, epigenetic modifications are reversible and thus able to dynamically modulate chromatin structure to activate or silence gene expression (Bannister & Kouzarides, 2011). Novel insights have highlighted the important role of non‐coding RNA transcripts in epigenetic gene regulation (Mattick & Makunin, 2006; Wei et al., 2017). The term non‐coding RNA (ncRNA) is commonly used for RNA that does not encode a protein, however, many of these RNAs do contain information and are functional. Non‐coding RNA helps control gene expression by attaching to coding RNA, along proteins that break down the coding RNA and prevent it from making proteins. Non‐coding RNA may also recruit proteins that modify histones to turn genes on or off by making them more or less accessible, respectively, to RNA‐making machinery. FIGURE 2.2 Overview of epigenetic modifications. Source: (Chaturvedi and Tyagi, 2014 / with permission of Elsevier). Alterations in epigenetic marks such as modification of the wrong gene or the failure to add a chemical group to a particular gene or histone, could lead to abnormal gene activity or inactivity (Casadesús & Noyer‐Weidner, 2013). Such epigenetic mechanisms could result in a vast spectrum of cell differentiations, morphogenesis, variability, and adaptability. Epigenetic modifications can directly cause disease, such as Beckwith Weidemann syndrome (BWS), an imprinting disorder caused by abnormal DNA methylation (Bakulski & Fallin, 2014). Epigenetics can also be the mechanism linking environmental exposures and disease, and classic example of this interaction is oxidative DNA damage caused by toxicants. Epigenetics may also influence the relationships between exposure and disease or genotype and disease via effect modification (i.e., exposure to air pollution and increased risk of cardiovascular disease). When targeting treatment, epigenetic factors can serve as useful biomarkers of disease status or disease subtype. Incorrect epigenetic marks can result in birth defects, childhood diseases, or diseases during a person’s lifespan. In this regard, epigenetic errors are associated with multiple NCDs, including cancer, cardiovascular, respiratory, and neurodegenerative diseases (Romani, Pistillo, & Banelli, 2015). Indeed, epigenetic modifications may be behind middle to late life functional declines. Early studies on epigenetic functions mainly focused on fetal development, aging, and cancer. As epigenetic research has progressed and epigenetic mechanisms have become better understood, possible epigenetic pathways have been implicated in complex diseases etiology, such as autoimmune diseases, inflammation, allergy, obesity, insulin resistance, type 2 diabetes (T2D), neurodegenerative diseases, autism spectrum disorders, bipolar disorder, and schizophrenia (Dupont et al., 2009; Ramsay, 2015). Epigenetic errors are also implicated in imprinting disorders, such as Silver–Russell syndrome (SRS) and Beckwith–Weidemann syndrome (BWS), for individuals conceived by assisted reproductive technologies (ART) (Peral‐Sanchez et al., 2021). ART conception is associated with an approximately two‐fold increased risk of preterm birth, low birth weight, being small for gestational age or perinatal mortality, some of which may be attributable to epigenetic variation induced in the periconceptional period. Genome‐wide DNA methylation profiling in cord blood and placenta revealed differences in the methylation status between ART and spontaneous conceiving populations (Novakovic et al., 2019). Across epigenomic sites, particular regions show change in methylation within the same person over time (Bakulski & Fallin, 2014). Furthermore, it was recently demonstrated that some epigenetic marks can be reversible, a trait that it is as challenging as it is promising (Bohacek & Mansuy, 2013; Foley et al., 2008). This characteristic makes the genome flexible to respond and adapt to external stimuli, such as nutrition, stress, exercise, pollutants, and drugs, since epigenetic modifications are dynamic and intertwined with environmental and lifestyle cues. What is beyond any doubt is that we are not born tabula rasa, as blank slates. Utero is the first environment that we are exposed to, and maternal stimuli can epigenetically modify the expression of our genes. It is well established that the environmental stimuli experienced during the first 1000 days of life, as early as pre‐conception, the nine months of pregnancy and the first two years of life, are transmitted to offspring and subsequent generations (Gabbianelli & Damiani, 2018) (Figure 2.3). Contrary to the common notion that the interaction between genes and the environment starts right after the birth, the fetal origins hypothesis was a scientific breakthrough in the late 1980s (Barker, 1995). Up until then, scientific research mainly focused on childhood environmental exposures and how these affected the risk of developing chronic diseases and mortality. On the other hand, Professor Barker, an innovating researcher, observed that a higher incidence of cardiovascular and infant mortality in regions of England and Wales was related to low birth weight, which was in turn associated with poor maternal nutrition and higher maternal mortality rates. These observations, along with the fact that offspring who weighed more at birth and were still breastfed 1 year later had lower death rates, led to Barker’s hypothesis of “the fetal and infant origins of adult disease”. According to this hypothesis, fetal undernutrition in middle to late gestation leads to disproportionate fetal growth, which predisposes them to certain diseases in adulthood. Hales and Barker later published the thrifty phenotype hypothesis, proposing that “the epidemiological associations between poor fetal and infant growth and the subsequent development of T2D and the metabolic syndrome result from the effects of poor nutrition in early life, which produces permanent changes in glucose‐insulin metabolism” (Hales & Barker, 2001). FIGURE 2.3 (a) Epigenetic reprogramming in the germ line and somatic cells. (b) Epigenetic reprogramming in embryos. Source: (Wallén et al., 2021 / MDPI / CC BY‐4.0). This influential theory aroused a great deal of research interest and activity in the field of developmental plasticity, and over the years, vast scientific data were documented that closely linked maternal obesity and gestational diabetes with several metabolic implications in offspring (Gabbianelli & Damiani, 2018). In addition, the Barker hypothesis set the basis for the developmental origins of health and disease hypothesis that “recognizes the broader scope of developmental cues, extending from the oocyte to the infant and beyond, and captures the concept that the early life environment has widespread consequences for later life” (Gillman, 2005; Koemel & Skilton, 2022). In fact, environmental exposures and lifestyle behaviors, from the prenatal period and on, seem to be the origin of diseases such as diabetes, cardiovascular diseases, obesity, and cancer. While one’s lifestyle clearly affects an individual’s health, vast epidemiological data emphasize that maternal and paternal lifestyle(s) can modify the risk of chronic diseases in subsequent generations (Donkin & Barrès, 2018). The ability of a given genotype to produce different phenotypes in response to different environments is plasticity and is part of the organism’s adaptability to environmental factors to provide the best chances to survive and reproduce (Bateson, Barker, et al., 2004). Our nature is designed to use placental, nutritional, and endocrine cues to set long‐term biological, mental, and behavioral alterations in response to internal and external stimuli (Kuzawa, 2005) (Figure 2.4). The window of developmental plasticity extends from conception to early childhood and adolescence and could be epigenetically transmitted to next generations. The epigenome, in utero and early in life, presents a high degree of plasticity. A wide spectrum of gestational cues, including diet, stress, toxins, and maternal lifestyle overall, significantly impacts fetal growth and epigenetically modulates the offspring’s phenotype and health (Koemel & Skilton, 2022). Maternal nutrition is an important genetic regulator, since nutritional exposures mediate fetal epigenetic modifications. Very interesting scientific data concerning the effect of nutrition during gestation arose from a classic epidemiological study during the Dutch famine of 1944–1945 (World War II), which demonstrated that individuals conceived during the famine were at a greater risk of CVD and metabolic disorders, such as obesity, diabetes, impaired glucose tolerance, and hyperlipidemia in adulthood (Lumey, 1992). Decades later, a significant reduction in DNA methylation in famine‐exposed offspring was observed at the promoter of the insulin‐like growth factor 2 (IGF2) gene, which regulates fetal development and growth. Most importantly, the Dutch famine data highlight that nutritional deprivation can have adverse effects on health beyond a person’s lifespan and leave a lasting epigenetic imprint on offspring. Similarly, epidemiological data from other famine studies (Hult et al., 2010; Li et al., 2010) demonstrate that adults exposed in utero or early in life to energy deficits had an increased risk for developing hypertension, glucose intolerance, and obesity compared to individuals conceived after the famine. FIGURE 2.4 Overview of epigenetic marks susceptible to remodeling with environmental stimuli. Source: (Donkin & Barrès, 2018 / with permission of Elsevier). Additionally, maternal malnutrition along with low‐protein diets from the pre‐implantation period to late lactation, is associated with the onset of metabolic diseases in adult offspring, which are mediated by epigenetic modifications (Koemel and Skilton, 2022; Peral‐Sanchez et al., 2021). Undernutrition during pregnancy may alter the fetal epigenome through reduced intake and bioavailability of protein, amino acids, and key micro‐nutrients involved in one‐carbon metabolism, such as folate, methionine, choline, betaine, vitamin B12, vitamin B6, antioxidants, and other bioactive compounds (Cai et al., 2021; Jankovic‐Karasoulos et al., 2021). The above‐mentioned nutrients are involved in one carbon metabolism and subsequently in DNA‐methylation process as co‐factors or methyl donors. More specifically, methionine, choline, and folate are substrates needed for the methylation process (methyl‐donor nutrients); the vitamins B2, B6, and B12 are co‐factors needed for methyltransferases, the enzymes involved in the methylation process (Kohil, Al‐Asmakh, Al‐Shafai, & Terranegra, 2021). Vitamin B6 serves as a coenzyme to serine hydroxymethyltransferase, the key enzyme in the folate cycle converting tetrahydrofolate (THF) to 5,10‐methyleneTHF. Riboflavin (vitamin B2) is a precursor for flavin adenine dinucleotides, which is a cofactor to methylenetetrahydrofolate reductase (MTHFR) in the conversion of 5,10‐methylene THF to 5‐methyl THF. Vitamin B12 is the coenzyme of methionine synthase, which catalyzes the reaction of homocysteine involved in the production of methionine from homocysteine and betaine (Figure 2.5) (Anderson, Sant, & Dolinoy, 2012). Folate‐mediated one‐carbon metabolism refers to a complex network of interconnected metabolic pathways, which ultimately result in a supply of methyl groups for DNA, RNA, or protein methylation. Folate, in particular, is a key source of the methyl group required for DNA and histone methylation, and sub‐optimal intake during gestation may alter methylation patterns that are vital for fetal development and the offspring’s health (Lintas, 2019). Most importantly, folate deficiency is correlated with methylation changes in brain tissue and may alter epigenetic marks implicated in neural tube defects, thus neurocognitive and neurobehavioral deficits (Joubert et al., 2016). FIGURE 2.5 Dietary methyl donors and one carbon metabolism. Source: (Anderson et al., 2012 / with permission of Elsevier). Interestingly, there is little evidence that nutritional interventions during early or mid‐pregnancy, like providing under‐nourished mothers with protein, energy, and micronutrient supplements, reduces the cardio‐metabolic disease risk in offspring. Data from animal studies suggest that it may be more crucial to intervene earlier in the pregnancy, when the developmental window is more critical, or even pre‐conception, to modify placentation and organogenesis (which occur mainly in the first trimester) as well as peri‐conceptional epigenetic changes (Fall & Kumaran, 2019). Excess fat and caloric consumption during gestation as well as a mother’s pre‐pregnancy obesity are also linked to epigenetic changes (Figure 2.6). Maternal metabolic disorders (e.g., poor glycemic control, hypertension) are associated with epigenetic alterations of the offspring too. Notably, genes that regulate energy and glucose metabolism as well as adipogenesis, leptin encoding, and transcription factors are controlled by epigenetic mechanisms (Elshenawy & Simmons, 2016). Maternal overnutrition may modify the expression of hypothalamic genes that can increase fetal and neonatal energy intake (Şanlı & Kabaran, 2019). Moreover, considering that lipids act as both transcriptional activators and signaling molecules, excess lipid exposure may regulate genes involved in lipid sensing and metabolism. It was suggested that the prevalence of an obese phenotype is closely related to epigenetic marks, originating from in utero stimuli and passing on through trans‐generational transmission (Heerwagen, Miller, Barbour, & Friedman, 2010). More specifically, leptin and insulin signals modify the development of eating behaviors by affecting the neuronal circuits in the hypothalamus. According to these neuroendocrine factors, increased fetal appetite, energy intake, and adiposity alter the food preferences and body composition after birth (Lawlor et al., 2006). Overall, maternal obesity and overnutrition can lead to permanent changes in metabolic and epigenetic mechanisms, and their offspring are predisposed to be overweight or with obesity themselves. Siblings born to mothers with obesity before and after the mother’s bariatric surgery had different obesity risks; the children born after weight loss had a lower risk of being overweight or with obesity (Şanlı & Kabaran, 2019). Therefore, considering the public health burden of obesity and metabolic diseases across generations, perinatal weight control interventions including lifestyle modifications through a healthy and balanced dietary pattern and exercise in mothers with obesity should be considered (Figure 2.7). FIGURE 2.6 Consequences of an early‐life exposure in an intrauterine obesogenic environment.
CHAPTER 2
Lifestyle and Epigenetics
INTRODUCTION
EPIGENETICS OVERVIEW
EPIGENETICS DEFINITION
EPIGENETIC MECHANISMS
EPIGENETICS AND DISEASE EPIDEMIOLOGY
THE BARKER HYPOTHESIS
EPIGENETICS IN UTERO AND MATERNAL LIFESTYLE
MATERNAL UNDERNUTRITION
MATERNAL OVERNUTRITION
DIETARY PATTERN AS AN EPIGENETIC MARK
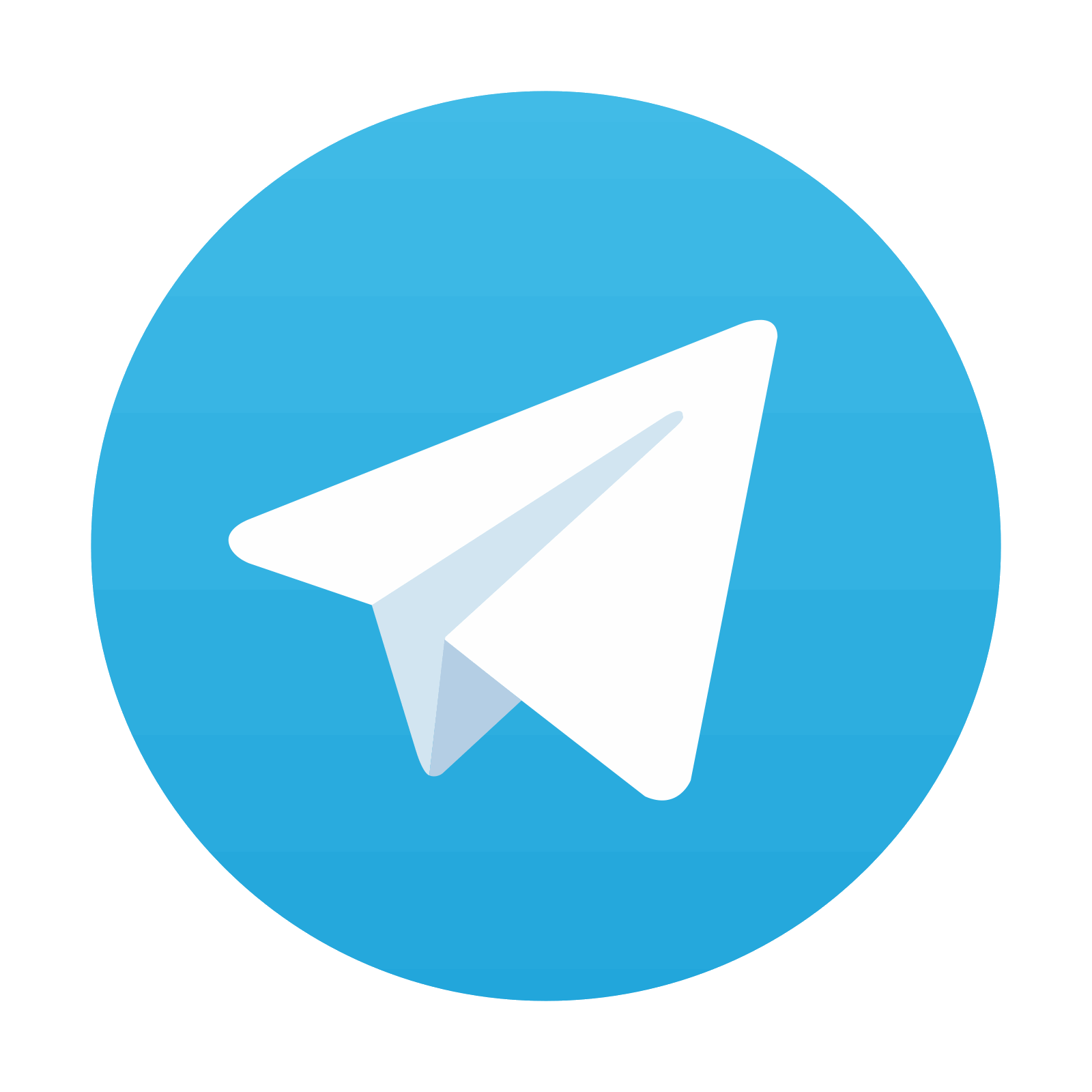
Stay updated, free articles. Join our Telegram channel
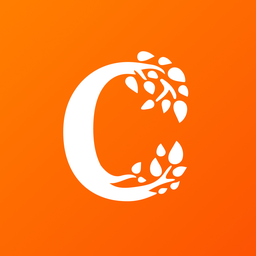
Full access? Get Clinical Tree
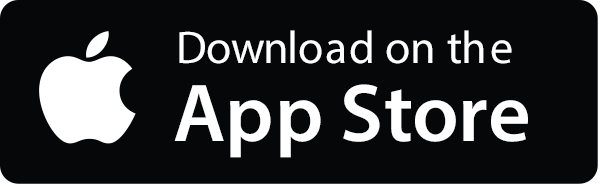
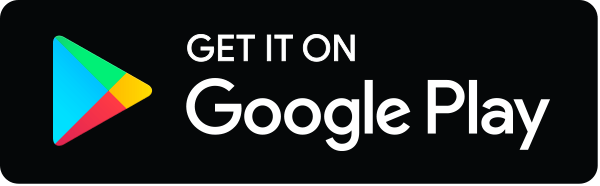