According to the International Classification of Sleep Disorders by the American Academy of Sleep Medicine, obstructive sleep apnea (OSA) belongs to a group of sleep‐related breathing disorders, along with central sleep apnea, sleep‐related hypoventilation, and sleep‐related hypoxemia disorder (International Classification of Sleep Disorders, 2014). It is a chronic pathological condition characterized by recurrent pauses of breathing during sleep due to obstruction of the upper airways, which leads to sleep disruption (Eckert & Malhotra, 2008; Jordan, McSharry, & Malhotra, 2014) (Figure 19.1). Specifically, in the context of OSA, narrowing or complete obstructions of the upper airways are observed in the pharyngeal region during sleep, when the tone of upper‐airway dilator muscles decreases normally and leads to temporary partial or complete cessation of breathing (respiratory events) despite respiratory effort. Breathing pauses are accompanied by a decrease in blood oxygen saturation (hypoxemia) and carbon dioxide retention (hypercapnia) that lead to sleep disruption (awakening) to restore the tone of the upper‐airway dilator muscles and normal airflow. However, after sleep restoration, upper‐airway obstructions reoccur and repetitive awakenings end up disrupting sleep architecture, duration, and quality (Eckert & Malhotra, 2008; Jordan et al., 2014). The clinical presentation of OSA includes symptoms that occur during both sleep and daytime. During sleep, OSA is characterized by a distinct pattern of snoring, which involves intervals of loud noises and respiratory efforts that alternate with intervals of silence due to complete breathing cessations. In most patients, snoring symptoms are usually present for many years, but the decision to undergo a sleep diagnostic procedure is usually made due to the aggravation of snoring frequency and/or intensity as reported by patients’ bedpartners. This aggravation is usually reported when alcohol has been consumed before bedtime or as a result of recent weight gain (Lurie, 2011; Stansbury & Strollo, 2015). Patients’ respiratory events can vary in severity, and in the case of severe OSA, they are maintained for several seconds before respiratory movements are observed and cause cyanosis due to hypoxemia. The end of respiratory events is usually accompanied by intense snoring and sounds that resemble “groans” or “squeals”, while awakenings are often accompanied by whole‐body movements, which can be sharp or even violent. The majority of patients are not fully aware of the breathing pauses and repetitive awakenings that occur during sleep but usually report insomnia symptoms and a sense of “restless” sleep (Lurie, 2011; Stansbury & Strollo, 2015). Nocturnal symptoms of the disease also include heavy sweating and nocturia, while early daytime symptoms manifest as fatigue, decreased rejuvenation, disorientation, mental sluggishness, lack of coordination, and headaches that often require analgesics (Lurie, 2011; Stansbury & Strollo, 2015). FIGURE 19.1 Schematic representation of the typical pathophysiological sequence that occurs in OSA. PCO2 = partial pressure of carbon dioxide; PO2 = partial pressure of oxygen. Polysomnography (PSG) is the gold‐standard method for OSA diagnosis (Epstein et al., 2009; Kapur et al., 2017; Qaseem et al., 2014). PSG is non‐invasive and consists of a simultaneous recording of multiple physiologic parameters related to sleep and wakefulness. The procedure is usually performed during night sleep in a laboratory (e.g., a sleep center) under the supervision of specialized medical personnel (sleep technologists and somnologists). In the context of the PSG, brain activity via electroencephalography, eye movements via electrooculography, muscle activity via electromyography, heart rate via electrocardiography, respiratory airflow via pressure transducers, respiratory effort via abdominal movement sensors, and blood oxygen levels via pulse oximetry are assessed during real sleep conditions, and all data are simultaneously recorded on a multi‐channel digital system (Figure 19.2) (Epstein et al., 2009; Kapur et al., 2017; Qaseem et al., 2014). After the procedure is completed, all recorded data are scored and analyzed according to the American Academy of Sleep Medicine guidelines (The AASM Manual for the Scoring of Sleep and Associated Events: Rules, Terminology and Technical Specifications, 2015), providing a detailed assessment of the patient’s sleep architecture and cardio‐respiratory function. With regard to sleep architecture, PSG analyses provide data on wake time, and time spent in non‐rapid eye movement (NREM) sleep, which consists of stage 1 (N1), stage 2 (N2), and stage 3 (N3) sleep as well as rapid eye movement (REM) sleep; for each sleep stage, the relative contribution to total sleep period is calculated as ([(time spent in sleep stage [min] ÷ total sleep period [min]) × 100]). Table 19.1 presents an overview of adult sleep staging and disturbances observed in OSA patients (Jordan et al., 2014). In general, human sleep consists of distinct sleep circles, each starting from a period of non‐REM sleep (N1 to N3) that is followed by a shorter period of REM sleep, which are repeated several times along with some arousals until the final awakening. Most patients with OSA spend more time awake, in N1, and N2 sleep and less time in N3 and REM sleep due to repetitive airway obstructions that lead to awakenings, disrupt normal sleep architecture, and prevent deeper sleep stages. Sleep latency (min), i.e., time from lights out to sleep onset, is also recorded, and sleep efficiency (%) is calculated as [(sleep time [min] ÷ time in bed [min]) × 100]. FIGURE 19.2 Schematic illustration of polysomnography. During the procedure, the following assessments are simultaneously performed: (1) electroencephalography, (2) electrooculography, (3) assessment of respiratory airflow, (4) mandible electromyography, (5) electrocardiography (heart rate), (6) assessment of body position, (7) assessment of respiratory effort (thoracic movements), (8) assessment of abdominal movements, (9) pulse oximetry (blood oxygen), and (10) lower limb electromyography. Source: (Sadek, Siyanbade, Abdulrazak, 2023) Table 19.1 Adult sleep staging and arousal scoring. REM = rapid eye movement; OSA = obstructive sleep apnea; EEG = electroencephalogram; EMG = electromyogram. Source: (Jordan et al., 2014). Respiratory events are classified as apneas (obstructive, central, or mixed) and hypopneas (The AASM Manual for the Scoring of Sleep and Associated Events: Rules, Terminology and Technical Specifications, 2015), depending on the severity of airflow reduction (Table 19.2). Based on the assessment of respiratory events, the apnea index (AI), the hypopnea index (HI), and the apnea‐hypopnea index (AHI) (total, non‐REM, and REM) are calculated as the number of corresponding respiratory events per hour of sleep [respiratory events (total number) ÷ sleep period (h)]. The respiratory disturbance index (RDI) can also be calculated; it is based on the number of apneas and hypopneas just like the AHI but also includes respiratory effort‐related arousals and usually receives slightly higher values compared to the AHI. Oximetry indices, such as average and minimum oxygen saturation (SaO2) are also recorded during PSG, and respiratory events accompanied by a decrease in SaO2 equal or greater than 4% during sleep, compared to SaO2 during wake period, are recorded to calculate the oxygen desaturation index (ODI) as [oxygen desaturation events (total number) ÷ sleep period (h)]. Electromyography of the anterior tibialis is also often performed to assess limb movements that might alter sleep stage or respiration and body position is monitored because of the position‐specific nature of OSA in many patients. Other parameters, such as average and highest heart rate, including episodes of bradycardia, asystole, tachycardia, atrial fibrillation, snoring, and any unusual or atypical behavioral events occurring during sleep and/or wakefulness are also recorded during PSG. Figure 19.3 provides an example of the signals recorded during an overnight PSG. Table 19.2 Adult respiratory event scoring. Source: (Jordan et al., 2014). According to the American Academy of Sleep Medicine International Classification of Sleep Disorders (International Classification of Sleep Disorders, 2014), the diagnosis of OSA requires the presence of signs‐symptoms (e.g., arousals accompanied by breathing difficulty or choking, snoring or witness of apneas during sleep, and daytime sleepiness) or co‐morbidities (e.g., hypertension, coronary heart disease, atrial fibrillation, congestive heart failure, stroke, diabetes mellitus, cognitive impairment, or psychiatric disorder), in combination with more than five, mainly obstructive, respiratory episodes per hour of sleep during a sleep study. Alternatively, OSA can be diagnosed in the presence of ≥15 respiratory events per hour of sleep even in the absence of associated signs‐symptoms or co‐morbidities. In clinical practice, the AHI, i.e., the number of apneas and hypopneas per hour of sleep, is the most important PSG measure, based on which OSA diagnosis and severity is evaluated (Sateia, 2014). An OSA diagnosis is established when the AHI receives values ≥5 events/h and the disease is further classified as mild (5–14 events/h), moderate (15–29 events/h) or severe OSA (≥30 events/h). OSA severity evaluation based on ODI and RDI values is similar to AHI classification, but these indexes are not widely used for OSA diagnosis and severity assessment in clinical practice. FIGURE 19.3 An example of the signals recorded during overnight polysomnography. The polysomnogram shows an obstructive apnea with cessation of airflow for more than 10 s despite persistent respiratory efforts shown on the chest and abdominal respiratory bands. The apnea is associated with arterial oxygen desaturation and is terminated by arousal from sleep. C4‐A1 = electroencephalogram; LOC = left electro‐oculogram; ROC = right electro‐oculogram; CHIN = chin electromyogram; CHEST = respiratory inductance plethysmography bands placed around the thorax; ABDM = respiratory inductance plethysmography bands placed around the abdomen; PNasal = airflow monitoring by nasal air pressure; Therm = airflow monitoring by thermal air sensor; SaO2 = arterial oxygen saturation; EKG = electrocardiogram; ABDM = abdomen. Source: (Jordan, McSharry, & Malhotra, 2014) Although an overnight attended PSG is the gold‐standard method for OSA diagnosis and provides definitive results, the procedure is expensive, time consuming, burdensome for most patients, and requires specialized personnel and equipment. Simplified home sleep studies using portable devices also provide basic information on sleep architecture and respiratory events and can alternatively be used for OSA diagnosis. Available evidence suggests that a home‐based diagnosis, if properly performed, is not inferior to a laboratory diagnosis and can be of great value for individuals with mobility problems who have difficulty accessing a sleep center or when an attended PSG is not available (Masa et al., 2011; Mulgrew, Fox, Ayas, & Ryan, 2007; Rosen et al., 2012). Even though the first reports of OSA date back to antiquity (Kryger, 1985), epidemiological data on the prevalence of the disease were not available until the early 1990s. The Wisconsin sleep cohort study, a prospective epidemiological study initiated in 1988 with the aim of investigating the prevalence and natural history of sleep‐related breathing disorders, is a landmark for the epidemiology of OSA (T. Young et al., 1993). According to analyses of 602 US men and women aged 30 to 60, the prevalence of OSA—defined as the presence of ≥5 apneas‐hypopneas/h based on PSG combined with increased daytime sleepiness—was 4% in men and 2% in women. Subsequent epidemiological studies in the USA, Europe, Australia, and Asia (Franklin & Lindberg, 2015; Punjabi, 2008; Senaratna et al., 2017) have shown that the prevalence of OSA in the general population, defined as AHI/ODI ≥5 events/h, ranges from 9% to 36%; disease rates are higher in men (from 13% to 33%) compared to women (from 6% to 19%) and increase with age, reaching 90% and 80% in older men and women, respectively. According to a comprehensive review of epidemiological studies examining global estimates of OSA prevalence published in 2019 (Benjafield et al., 2019), 936 million adults aged 30 to 69 years have mild‐to‐severe OSA and 425 million have moderate‐to‐severe disease; the number of affected individuals was highest in China, followed by the USA, Brazil, and India. It is worth mentioning, however, that despite the widespread recognition of OSA as a major public health problem, available estimates of its prevalence probably underestimate its true extent, given that a large part of the general population (approximately 20%) experiences the respiratory manifestations of the disease in the absence of accompanying symptoms or co‐morbidities that usually lead to diagnostic sleep testing. Moreover, a large proportion of patients, even those with symptoms or those at increased risk for co‐morbidities, remain undiagnosed due to the practical difficulties of PSG. OSA has been traditionally considered a disease of abnormal upper‐airway anatomy and neuromuscular pathology, in which abnormal craniofacial structure or excess body fat decreases the size of the pharyngeal airway lumen and, combined with upper‐airway muscle dysfunction, leads to an increased likelihood of pharyngeal collapse during sleep (Eckert & Malhotra, 2008; Horner, 2008; Patil, Schneider, Schwartz, & Smith, 2007). The pharynx is a common organ for the respiratory system and the gastrointestinal tract, and it is involved in many vital body functions, such as swallowing, speaking, and breathing. It is unsupported by bones or cartilaginous tissue, but it contains several groups of muscles and soft tissues, along with a section that can be closed, which extends from the hard palate to the larynx (Ayappa & Rapoport, 2003). This ability of the pharynx to close is essential for speech and swallowing while awake, but it also provides the grounds for an undesirable obstruction under certain conditions during sleep. During wakefulness, the upper airway is held open by the high activity of the numerous upper‐airway dilator muscles. After the onset of sleep, when muscle activity is normally reduced, the airway is susceptible to obstruction, especially in the presence of a detrimental upper‐airway anatomy. The size of the upper airways in the pharyngeal region depends on many factors, as the pharyngeal lumen is surrounded lengthwise by bones, such as the nasal bones, jaw bones, and the hyoid bone, as well as soft tissues, such as the tongue, the soft palate, the tonsils, the epiglottis, fat particles, and blood vessels. In general, a disproportionately high proportion of soft tissue mass, relative to the space available from the skeletal structures surrounding the pharynx, favors its obstruction (C. M. Ryan & Bradley, 2005). Patients with OSA often present with disorders of the skeletal structures surrounding the pharyngeal cavity, such as hypoplasia and/or dislocation of the jaws and displacement of the hyoid bone as well as enlargement of the soft tissues surrounding the pharynx, such as the thickening of the lateral walls of the pharynx (secondary to the accumulation of fat in the area, swelling of the pharyngeal mucosa due to vascular congestion or inflammation, or swelling of the veins in the area in the presence of fluid accumulation due to heart failure or chronic kidney disease) or the swelling of the soft palate and the tonsils (Eckert & Malhotra, 2008; Horner, 2008; Patil et al., 2007). In addition to this detrimental upper‐airway anatomy, compared to healthy subjects, patients with OSA also experience a greater reduction in genioglossus muscle activity, the most important upper‐airway dilator muscle, and its response to local stimuli during sleep. Upper‐airway muscle fatigue is also thought to be involved in OSA pathogenesis, caused by chronic muscle trauma and inflammation due to continuous low‐frequency vibrations of snoring and recurrent hypoxia (Eckert & Malhotra, 2008; Horner, 2008; Patil et al., 2007). Therefore, although healthy individuals experience only a small degree of respiratory instability at the onset of sleep, a person with a detrimental upper respiratory tract anatomy who relies more on muscle tone to support respiration is particularly prone to developing OSA when the function of the upper‐airway dilator muscles is compromised. Although upper‐airway anatomy and muscle function are crucial for the development of OSA, accumulated research over the last decades has also identified several non‐anatomical and non‐neuromuscular factors that contribute to OSA pathophysiology in patients with apparently normal upper‐airway anatomy and very responsive upper‐airway dilator muscles. These factors include the instability of the central respiratory control system, increased tendency to wake up (low arousal threshold), and low lung volume (Eckert & Malhotra, 2008; Horner, 2008; Patil et al., 2007). In addition, the close relationship between OSA and cardio‐metabolic diseases has led the scientific community to reconsider its traditional view as merely an anatomical disease of the upper respiratory system and investigate cardio‐metabolic factors as potential pathogenetic mechanisms. In this context, the landmark theory of Vgontzas et al. (Vgontzas, Bixler, & Chrousos, 2003, 2005; Vgontzas, Gaines, Ryan, & McNicholas, 2016) suggests that cardio‐metabolic disorders in the context of the metabolic syndrome, i.e., central obesity, insulin resistance, dyslipidemia, and hypertension are implicated in OSA pathogenesis. In particular, while the majority of researchers argue that inflammation and oxidative stress resulting from intermittent hypoxia in the context of OSA lead to cardio‐metabolic morbidity, Vgontas et al. (Vgontzas et al., 2003, 2005; Vgontzas et al., 2016) suggest that the pathophysiology of the metabolic syndrome actually precedes the onset of OSA, with central fat deposition triggering upper‐airway dysfunction through insulin resistance, inflammation and oxidative stress, and that OSA should be essentially be considered as the manifestation of the metabolic syndrome in the respiratory system of obese individuals. This hypothesis is based on evidence that: i) obesity, and diseases in which insulin resistance and inflammation play a central role, such as diabetes mellitus, are associated with symptoms of OSA (e.g., daytime sleepiness) in the absence of a formal OSA diagnosis; ii) treatment of OSA‐related respiratory disorders is not accompanied by benefits in glucose metabolism, inflammation, and oxidative stress, suggesting that these disorders precede OSA rather than manifest as a result of the disease; iii) exercise can reduce OSA severity through improvements in inflammation and insulin resistance in the absence of weight loss; iv) the presence of the metabolic syndrome is associated with sarcopenia and skeletal muscle dysfunction and may consequently contribute to upper‐airway dilator muscle dysfunction; v) elevated leptin levels, a key feature of obesity, can disrupt central respiratory control; vi) the prevalence of OSA and the metabolic syndrome has a similar age distribution in the general population, reaching a maximum in middle age, a fact that supports their causal relationship; and vii) inflammation is present in patients with OSA regardless of the presence of obesity (Vgontzas et al., 2003, 2005; Vgontzas et al., 2016). Risk factors for developing OSA resemble those of cardiovascular diseases and include increased age, obesity, male gender, smoking, and heavy alcohol consumption (Gharibeh & Mehra, 2010; Punjabi, 2008). OSA also exhibits a hereditary pattern, with up to 35% of the variation in disease severity being attributed to genetic factors regardless of age and body‐weight status (Gharibeh & Mehra, 2010; Punjabi, 2008). Among modifiable risk factors, obesity has emerged as the most important factor leading to sleep‐disordered breathing and the progression of OSA. Epidemiological studies suggest that being overweight occurs in approximately 60% of patients undergoing diagnostic sleep testing. The Wisconsin sleep cohort study was the first large‐scale prospective study to test longitudinal associations between weight change and OSA indices (Peppard, Young, Palta, Dempsey, & Skatrud, 2000). In a sample of 690 participants from Wisconsin, USA who were followed for 4 years, compared to weight‐stable conditions, a 5%, 10%, and 20% weight gain predicted a 15%, 32%, and 70% increase in the AHI, respectively, while weight loss was associated with analogous decreases in the AHI (Peppard et al., 2000). Similar observations were subsequently reported by the sleep heart health study among 2968 middle‐aged men and women from several US communities during a 5‐year follow‐up period, compared to weight‐stable individuals; those who gained weight experienced increases in the RDI in a dose‐response manner and vice versa (Newman et al., 2005). Several case‐control studies have also revealed a significant positive association between the presence of overweight/obesity and odds of OSA both in children and adults (Dong et al., 2020). Results of available interventional studies reinforce the epidemiological observations, as weight loss has been shown to improve the severity of OSA in most patients (these data are discussed below in detail). The involvement of obesity in the pathophysiology of OSA can be explained through several mechanisms, including: i) central fat accumulation, mainly in abdominal viscera and around the neck, which leads to increased mechanical load on upper airways; ii) increased leptin resistance, which can impair central respiratory control and lead to abnormal hypercapnic ventilatory response; iii) expression of pro‐inflammatory cytokines, which negatively impact upper‐airway neuromuscular control; and iv) a state of increased oxidative stress, which reduces the force‐generating capacity of upper‐airway muscles (Figure 19.4) (Dobrosielski, Papandreou, Patil, & Salas‐Salvado, 2017). It is also worth noting that, regardless of total body mass, an increasing number of studies highlight the potential aggravating role of upper body fat deposition in the development of OSA, in particular visceral adipose tissue (Kritikou et al., 2013; Vgontzas et al., 2000), a metabolic active tissue associated with cardio‐metabolic disorders, and the adipose tissue that accumulates in the area of the upper airways (Mortimore, Marshall, Wraith, Sellar, & Douglas, 1998; Pahkala, Seppa, Ikonen, Smirnov, & Tuomilehto, 2014), which, as mentioned above, contributes to the thickening of the lateral walls of the pharynx. FIGURE 19.4 Schematic diagram indicating the possible mechanisms linking obesity/central obesity with OSA development. CNS = central nervous system; OSA = obstructive sleep apnea. Source: (Dobrosielski et al., 2017). Disruption of the normal sleep architecture is the main short‐term complication of OSA. As shown in Table 19.1, sleep is divided into NREM and REM sleep, while NREM sleep is further subdivided into three stages (N1 to N3), which represent the successive transition from lighter to deeper sleep (Kales, Rechtschaffen, University of California, Brain Information, & Network, 1968). Under normal conditions, in adults, N1 sleep accounts for 5%, N2 sleep for 50% to 65%, N3 sleep for 15% to 20%, and REM sleep for 15% to 20% of total sleep (Karacan, 1970). In OSA, these patterns are significantly disturbed, with the duration of N1 and N2 sleep being increased, and the duration of N3 (slow‐wave sleep) and REM sleep being significantly reduced (Jordan et al., 2014) due to repeated interruptions of breathing leading to awakenings. Given the significant disruption of normal sleep architecture, OSA is typically characterized by a wide variety of daytime symptoms, including sleepiness, fatigue, low vitality, inability to concentrate, impaired reflexes, and memory deficits (Bucks, Olaithe, & Eastwood, 2013; Wallace & Bucks, 2013). The most prevalent daytime symptom is sleepiness, which is evident in most patients when at a relaxed state, e.g., after lunch, when reading, or watching TV, and in severe OSA manifests as involuntary sleep episodes during social circumstances (e.g., theater or cinema), work environment (e.g., meetings), and driving (Black, 2003; Pagel, 2008). OSA daytime symptoms collectively lead to reduced functionality and productivity that can negatively affect personal relationships or work efficiency and contribute to psychological disorders (Brown, 2005), while most patients are prone to self‐injuries and accidents (Ellen et al., 2006; Rodenstein, 2009). Besides daytime symptomatology, OSA is strongly linked to cardiovascular morbidity. This association is partly because OSA and cardiovascular disease share common risk factors, as discussed earlier in this chapter. However, OSA has also been suggested as an independent risk factor for cardiovascular disorders, a theory based on its adverse effects on the cardiovascular system, which is exposed to a vicious cycle of hemodynamic, oxidative, inflammatory, and metabolic disorders during sleep‐disordered breathing (Figure 19.5) (Bradley & Floras, 2009). Overall, the literature agrees that OSA is a major cause of cardiovascular morbidity and mortality and not just a respiratory disorder (Bradley & Floras, 2009; Kasai, Floras, & Bradley, 2012; Sanchez‐de‐la‐Torre, Campos‐Rodriguez, & Barbe, 2013). Several health organizations, such as the European and the American Heart Association, recognize OSA as a modifiable risk factor for cardiovascular disease (Piepoli et al., 2016; Somers et al., 2008). Intermittent hypoxia plays a central role in the pathogenesis of cardio‐metabolic complications of OSA, due to its effects on the central nervous system and its various oxidative, inflammatory, and metabolic consequences (Figure 19.6) (Bonsignore, McNicholas, Montserrat, & Eckel, 2012; Bradley & Floras, 2009). During normal sleep, the parasympathetic activity of the central nervous system outweighs the sympathetic one; however, intermittent hypoxemia and the subsequent awakenings in the context of OSA cause an abnormal increase in sympathetic activity during sleep, which is followed by an increase in catecholamine levels. Hyperactivity of the sympathetic nervous system is accompanied by an increase in blood pressure, a disorder that often persists during the day and, in the long term can lead to the establishment of hypertension. This increase in blood pressure levels, combined with the fluctuating intrathoracic pressure due to respiratory effort and the reduction of oxygen supply to body tissues due to hypoxemia, significantly impairs myocardial and vascular function and increases the risk of cardiac arrhythmias, heart failure, and ischemic stroke (Bonsignore et al., 2012; Bradley & Floras, 2009). In addition, recurrent episodes of oxygen desaturation and re‐oxygenation within OSA lead to the production of oxygen free radicals and the establishment of an environment of increased oxidative stress, which in turn results in macromolecular peroxidation, changes in cellular homeostasis, and increased expression of inflammatory genes controlled by the nuclear factor κB. This change in the expression of inflammatory genes results in an increased production of inflammatory markers, mainly tumor necrosis factor‐α, C‐reactive protein, interleukin‐6, and interleukin‐8 as well as a decreased production of anti‐inflammatory markers, such as adiponectin. The combination of sympathetic nervous system hyperactivation, oxidative stress, and chronic subclinical inflammation leads to long‐term endothelial dysfunction and arterial stiffness, factors that play a central role in the establishment and development of atherosclerosis, the background of cardiovascular disease (Bonsignore et al., 2012; Bradley & Floras, 2009). Finally, chronic sleep deprivation or poor sleep quality, combined with oxidative stress and chronic inflammation, also adversely affect the body’s metabolic homeostasis, triggering insulin resistance. Insulin resistance, combined with obesity, which is present in the majority of patients with OSA, lead to increased adipose tissue lipolysis and increased availability of free fatty acids to the circulation, which are stored ectopically in the liver and lead to hepatic steatosis, increased de novo lipogenesis, and eventually to atherogenic dyslipidemia, which manifests as elevated triglycerides (TG), total cholesterol (TC), low‐density lipoprotein cholesterol (LDL‐cholesterol), and very low‐density lipoprotein cholesterol (VLDL‐cholesterol), and low levels of high‐density lipoprotein cholesterol (HDL‐cholesterol) (Bonsignore et al., 2012; Bradley & Floras, 2009). FIGURE 19.5 Pathophysiological effects of OSA on the cardiovascular system. PNA = parasympathetic nervous system activity; PO2 = partial pressure of oxygen; PCO2 = partial pressure of carbon dioxide; SNA = sympathetic nervous system activity; HR = heart rate; BP = blood pressure; LV = left ventricle. Source: (Bradley & Floras, 2009). FIGURE 19.6 Functional consequences of visceral obesity, OSA and intermittent hypoxia in adipocytes, skeletal muscle, the liver, and the vessel wall. TG = triglycerides; FFA = free fatty acid; Apo = apolipoprotein; VLDL = very low‐density lipoproteins; sdLDL = small dense low‐density lipoproteins; HDL = high‐density lipoproteins; IH = intermittent hypoxia; OSA = obstructive sleep apnea. Source: (Bonsignore et al., 2012). The hemodynamic, oxidative, inflammatory, and metabolic complications of OSA lead to an overall increased cardio‐metabolic risk. This hypothesis is supported by numerous cross‐sectional epidemiological studies showing an increased prevalence of cardiovascular diseases in patients with OSA and vice versa (Floras, 2018; Sarkar, Mukherjee, Chai‐Coetzer, & McEvoy, 2018) as well as prospective epidemiological studies that reveal a strong positive relationship between the presence and severity of OSA and the risk of hypertension, diabetes mellitus, arrhythmias, coronary heart disease, heart failure, stroke, cardiovascular, and total mortality (Hou et al., 2018; Wang, Bi, Zhang, & Pan, 2013; Xie, Zhu, Tian, & Wang, 2017). Treatment modalities for OSA aim to reverse the underlying pathology, i.e., to prevent the obstruction of the upper airways during sleep, and include: i) the administration of positive airway pressure (PAP), which is currently the first‐line treatment for all OSA patients; ii) weight loss in overweight/obese patients (a detailed overview of data on weight loss and OSA management is presented later in this chapter); and iii) the use of oral devices or surgical procedures in patients with severe upper respiratory tract anatomical disorders (Gaisl, Haile, Thiel, Osswald, & Kohler, 2019; Patil et al., 2019; Veasey et al., 2006). Positional therapy, i.e., avoidance of sleeping in the supine position in which the upper airway becomes more susceptible to obstruction due to gravity, and sleep hygiene techniques (e.g., maintaining a regular bedtime schedule, avoidance of sleeping pills, avoidance of alcohol, caffeine, or other stimulants before sleep, consumption of a light dinner to prevent gastrointestinal symptoms that can further disrupt sleep, etc.) are also used as secondary measures for OSA management (Gaisl et al., 2019; Patil et al., 2019; Veasey et al., 2006). Regarding pharmacotherapy, to date, there is no established pharmacological treatment for OSA, and specific medications are individually prescribed to patients in the presence of co‐morbidities (e.g., hypolipidemic agents in case of dyslipidemia). CPAP was introduced as a therapeutic approach for OSA in 1981 (Sullivan, Issa, Berthon‐Jones, & Eves, 1981) and ever since has been recommended as the first‐line treatment for the disease (Epstein et al., 2009; Qaseem et al., 2013). It is a form of PAP ventilation in which a constant level of pressure greater than atmospheric is continuously applied to the upper respiratory tract of a patient during sleep, to prevent upper airway collapse and respiratory events (Figure 19.7). CPAP is currently prescribed as long‐life standard care to most patients with OSA in clinical practice and has been found efficient in minimizing the frequency of sleep‐disordered breathing, reducing OSA symptoms (e.g., daytime sleepiness), and improving patient quality of life compared to control therapies. However, it is also characterized by several limitations. Low adherence to CPAP, typically defined as use for <70% of nights and <4 h/night, has been documented in up to 80% of patients with OSA (Bakker, Weaver, Parthasarathy, & Aloia, 2019; Giles, 2015; Rotenberg, Murariu, & Pang, 2016; Sawyer et al., 2011), while in some cases its discontinuation even for one night leads to the recurrence of OSA manifestations, i.e., respiratory events and daytime symptoms (Kribbs et al., 1993; Rossi, Schwarz, Bloch, Stradling, & Kohler, 2014; Sforza & Lugaresi, 1995; L. R. Young et al., 2013). Moreover, evidence of the cardio‐metabolic benefits of CPAP is currently lacking. According to meta‐analyses of the available well‐designed randomized controlled clinical trials, CPAP therapy does not seem to improve glucose metabolism indices, lipidemic profile, low‐grade inflammation biomarkers, or cardiovascular morbidity and mortality (Feng, Zhang, & Dong, 2015; Jullian‐Desayes et al., 2015; Yu et al., 2017), a fact that significantly limits its value as the sole treatment for most high cardiovascular risk OSA patients. Finally, CPAP has been shown to promote a modest but significant increase in body weight (possibly due to reversing chronic hypoxemia, which has been associated with increases in resting energy expenditure), further aggravating overweight/obesity in patients with OSA initiated on CPAP therapy (Drager et al., 2015; Hoyos et al., 2019). These limitations challenge the value of CPAP as the sole treatment for OSA and highlight the importance of novel therapies for the disease management. In this context, behavioral interventions toward weight loss through beneficial changes in dietary and physical habits that do not require sophisticated equipment and can be feasible in all healthcare services can constitute a realistic and efficient plan for the management of OSA in clinical practice (Hudgel et al., 2018), as presented in detail later in this chapter. The use of oral devices and surgical procedures are alternative treatments for some patients with OSA, especially those with a detrimental upper‐airway anatomy. Oral appliances aim to move the lower jaw forward or to restore the correct position of the tongue, with the ultimate goal of maintaining the accessibility of the upper airways during sleep through a geometrically favorable air flow geometry (A. T. Ng, Gotsopoulos, Qian, & Cistulli, 2003; C. F. Ryan, Love, Peat, Fleetham, & Lowe, 1999). Most clinical trials to date suggest that these devices can improvement the severity of the OSA and its symptoms, albeit to a lesser extent than that achieved by application of CPAP therapy (Lim, Lasserson, Fleetham, & Wright, 2006; Sharples et al., 2016). Possible side effects include pain in face muscles, temporomandibular joints and teeth, excessive salivation, xerostomia (dry mouth), and gum irritation (Ferguson, Cartwright, Rogers, & Schmidt‐Nowara, 2006). The surgical treatment of OSA aims to increase the cross‐sectional area of the upper airways by correcting the aggravated anatomy of the upper respiratory tract, either by removing unnecessary/enlarged parts of the soft tissues of the pharynx or by correcting the skeletal structures that surround it. Various surgical procedures have been investigated in the context of OSA management, the main one being uvulopalatopharyngoplasty, i.e., the removal of the tonsils, the uvula, and part of the soft palate, which was first applied in 1981 (Fujita, Conway, Zorick, & Roth, 1981); other techniques have also been developed over time and include functional rhinoplasty, septoplasty, turbinoplasty, palatal surgery, and orthognathic surgery (Tanna et al., 2016). Overall, the implementation of surgery to treat OSA has been shown to lead to moderate improvements in disease severity, especially in patients with severe structural abnormalities of the upper respiratory tract; however, these improvements are not greater than those resulting from CPAP treatment, while improvements in daytime sleepiness and patients’ quality of life has not been confirmed by all available studies (Back et al., 2009; Ceylan et al., 2009; Ferguson, Heighway, & Ruby, 2003; Larrosa et al., 2004; Li, Wang, Lee, Chen, & Fang, 2006; Stuck, Sauter, Hormann, Verse, & Maurer, 2005; Woodson, Steward, Weaver, & Javaheri, 2003). FIGURE 19.7 Mechanism of upper‐airway occlusion and its prevention by CPAP. When the patient is awake (left), muscle tone prevents collapse of the airway during inspiration. During sleep, the tongue and soft palate are sucked against the posterior oropharyngeal wall (middle). CPAP with low pressure provides a pneumatic splint and keeps the airway open (right). CPAP = continuous positive airway pressure. Source: (Sullivan et al., 1981). Data on the association between long‐term dietary habits and the presence or severity of OSA are so far scarce, and most studies have focused on specific dietary nutrients. Available epidemiological case‐control studies have reported a higher intake of saturated fatty acids and a lower intake of carbohydrates, dietary fiber, thiamine, folic acid, vitamin E, and potassium among patients with OSA and/or other sleep disorders (e.g., chronic insomnia) compared to healthy controls (Stelmach‐Mardas, Mardas, Iqbal, Kostrzewska, & Piorunek, 2017; Tan et al., 2015). In addition, epidemiological cross‐sectional studies among OSA patients with varying degrees of disease severity have revealed higher intakes of protein, total fat, cholesterol, and saturated fatty acids in patients with severe OSA compared to those with mild or moderate disease (Vasquez, Goodwin, Drescher, Smith, & Quan, 2008). The levels of docosahexaenoic acid (DHA) in red blood cell membranes have also been negatively correlated with OSA severity after adjustment for habitual fish consumption and the use of omega‐3 fatty acid supplements (Ladesich, Pottala, Romaker, & Harris, 2011); although there are no data linking dietary omega‐3 fatty acid intake to the presence and/or severity of OSA, it is possible that omega‐3 fatty acids can alleviate OSA severity due to their strong anti‐inflammatory properties and contribute to a more favorable cardio‐metabolic profile, a hypothesis that remains to be confirmed (Scorza et al., 2013). Besides individual nutrients, OSA severity has been positively correlated to the consumption of red meat, meat products, and refined grains and negatively correlated to the consumption of whole grains and total dietary quality assessed through the alternative healthy eating index (Kechribari et al., 2020; Kechribari et al., 2021; Reid et al., 2019). Although the aforementioned data come from epidemiological studies, which cannot establish causal relationships, it is possible that adherence to prudent dietary patterns characterized by a high consumption of fruits, vegetables, whole grains, legumes, nuts, vegetable oils (e.g., olive oil), and fish, and a low consumption of animal‐based products, such as red meat, cold cuts, butter, and processed foods, can offer protection against the development and progression of OSA and should be encouraged as a strategy for preventing sleep‐disordered breathing in the general population. Beyond its potential impact on respiratory events, the adoption of a heathy dietary pattern can also act toward cardio protection and the prevention of OSA‐related cardio‐metabolic morbidity. Despite the lack of evidence on the role of long‐term dietary habits as a risk factor for OSA, nutrition has an important role in the management of sleep‐disordered breathing. Given the close relationship between OSA and obesity, dietary modifications toward weight loss have long been considered essential for most patients with OSA and are currently advocated as an ancillary treatment for the disease. Randomized controlled clinical trials (Foster et al., 2009; Johansson et al., 2011; Johansson et al., 2009; Kemppainen et al., 2008; Kuna et al., 2013; Nerfeldt, Nilsson, Udden, Rossner, & Friberg, 2008; S. S. Ng et al., 2015; Sahlman et al., 2012; Schwartz et al., 1991; Smith, Gold, Meyers, Haponik, & Bleecker, 1985; H. Tuomilehto et al., 2010; H. Tuomilehto et al., 2013; H. P. Tuomilehto et al., 2009) comparing the effects of an intensive weight‐loss intervention (usually a prescription of very low‐ or low‐calorie diets combined with lifestyle counseling or counseling toward reduction in energy intake in individual or group sessions without a standard dietary plan) versus standard care (simple dietary/lifestyle advice or no active intervention) on OSA management have consistently highlighted the benefits of weight loss in improving disease severity (e.g., decline in the AHI) and symptomatology (e.g., alleviation of daytime sleepiness). Meta‐analyses of these studies reveal that an intensive weight‐loss intervention can lower the AHI by approximately 15 events/h compared to standard care (Anandam, Akinnusi, Kufel, Porhomayon, & El‐Solh, 2013; Mitchell et al., 2014), which is considered a clinically meaningful reduction especially for patients with mild‐to‐moderate OSA. Uncontrolled clinical trials (Aubert‐Tulkens, Culee, & Rodenstein, 1989; Barnes, Goldsworthy, Cary, & Hill, 2009; Dobrosielski, Patil, Schwartz, Bandeen‐Roche, & Stewart, 2015; Fujii, Miyamoto, Miyamoto, & Muto, 2010; Hakala, Maasilta, & Sovijarvi, 2000; Hakala, Mustajoki, Aittomaki, & Sovijarvi, 1995; Hernandez et al., 2009; Kajaste, Telakivi, Mustajoki, Pihl, & Partinen, 1994; Kansanen et al., 1998; Kiselak, Clark, Pera, Rosenberg, & Redline, 1993; Kulkas et al., 2015; Lojander, Mustajoki, Ronka, Mecklin, & Maasilta, 1998; Nahmias, Kirschner, & Karetzky, 1993; Nerfeldt, Nilsson, Mayor, Udden, & Friberg, 2010; Nerfeldt, Nilsson, Mayor, et al., 2008; Noseda, Kempenaers, Kerkhofs, Houben, & Linkowski, 1996; Papandreou, Hatzis, & Fragkiadakis, 2015; Papandreou, Schiza, Bouloukaki, et al., 2012; Papandreou, Schiza, Tzatzarakis, et al., 2012; Pasquali et al., 1990; Rajala et al., 1991; Sampol et al., 1998; Suratt, McTier, Findley, Pohl, & Wilhoit, 1987; H. P. Tuomilehto et al., 2009) have also shown that weight loss can promote significant improvements in respiratory events, oximetry indices, sleep architecture, and quality of life among overweight and obese OSA patients with varying degrees of disease severity. In most studies, baseline OSA severity and the degree of weight loss were the strongest predictors of improvement. Although the dose‐response relationship between the magnitude of weight loss and OSA management requires further investigation, most clinical trials have revealed that the greater the weight loss, the greater the improvements in OSA severity, with patients achieving a ≥5% to 10% weight loss exhibiting the greatest benefits (Georgoulis et al., 2022; Kulkas et al., 2015; Papandreou et al., 2015; H. P. Tuomilehto et al., 2009). This is in line with current guidelines for obesity management that suggest a 5% to 10% weight loss over 6 months as a realistic goal sufficient to produce health benefits in most overweight/obese individuals; an even greater degree of weight loss of ≥10% may be suitable for patients with higher degrees of obesity, however, even a mild weight loss of 3% to 5% is considered beneficial for health, especially for obese patients at high risk of, or with, established obesity‐related chronic diseases and could constitute an initial goal for OSA patients who struggle with long‐term weight regulation (Jensen et al., 2014; Stegenga, Haines, Jones, Wilding, & Guideline Development, 2014; Yumuk et al., 2015). A similar dose‐response pattern has been reported for weight loss and improvements in cardio‐metabolic disorders, with which OSA frequently co‐occurs or shares a common pathogenetic background, such as type 2 diabetes mellitus, dyslipidemia, hypertension, and non‐alcoholic fatty liver disease (D. H. Ryan & Yockey, 2017). However, not all scientific data support this dose‐response relationship. For example, Dixon et al. (Dixon et al., 2012) reported that laparoscopic adjustable gastric banding did not result in a statistically greater reduction in 2‐year AHI levels compared to conventional weight‐loss therapy through dietary modification, despite an almost 6‐fold greater weight loss among 60 obese participants with a baseline AHI of ≥20 events/h. They also observed a strong positive correlation between weight loss and AHI decline in the conventional weight‐loss group but not in the bariatric surgery group. The authors concluded that there was a non‐linear link between weight loss and OSA severity with considerable individual effect variability as well as a complex, rather than pure mechanical load, pathogenesis of OSA in obese individuals (Dixon et al., 2012). It is therefore possible that although mild‐to‐moderate weight loss (5‐15%) is associated with proportional improvements in the AHI, the dose‐response relationship eventually reaches a plateau with limited additional benefit from further weight loss. Whether lifestyle‐induced weight loss and CPAP treatment are similarly effective for OSA management has so far been investigated in a small number of studies. A randomized clinical trial (Chakravorty, Cayton, & Szczepura, 2002) comparing a non‐intensive lifestyle modification program (sleep hygiene, smoking cessation, stress management, avoidance of alcohol, and simple advice on weight loss and physical activity) with CPAP treatment revealed benefits from both interventions in sleep architecture and daytime sleepiness; the lifestyle intervention resulted in greater weight loss, while CPAP therapy led to greater improvements in the AHI, oxygen saturation, and quality of life. A few more randomized clinical trials (Ballester et al., 1999; Lam et al., 2007; Monasterio et al., 2001) have compared the combination of a weight‐loss intervention with CPAP versus a weight‐loss intervention alone in OSA and showed the superiority of the combination therapy in reducing OSA severity and symptoms; however, the weight‐loss intervention used in these studies involved a generalized, non‐intensive lifestyle program that included simple sleep hygiene tips and weight‐loss recommendations, which served as a control treatment and probably had little effect on OSA. More recent data from well‐designed, randomized controlled clinical trials (Georgoulis, Yiannakouris, Kechribari, et al., 2021; Helena, Margareta, Eva, & Pernilla, 2014; Igelstrom, Asenlof, Emtner, & Lindberg, 2018) have shown that, compared to CPAP alone, its combination with an intensive weight‐loss intervention (counseling sessions targeting weight loss, prudent dietary habits, and adequate physical activity) is superior in improving anthropometric indices, lifestyle habits, and OSA severity and symptomatology, which highlights the necessity of weight loss on top of CPAP prescription for overweight/obese patients with OSA. Besides improvements in respiratory lesions, recent research has also focused on the cardio‐metabolic benefits of weight loss in OSA. In the clinical trial of Chirinos et al. (Chirinos et al., 2014) published in 2014, 181 patients with AHI ≥15 events/h were randomly allocated to three interventions, i.e., CPAP treatment, weight loss (hypocaloric diet), or a combination of both, for 24 weeks. The study revealed benefits in arterial pressure in all three study groups, but only patients receiving the weight‐loss regimen exhibited improvements in lipidemic profile indices, triglycerides, LDL‐cholesterol, and C‐reactive protein as well as an increase in insulin sensitivity as assessed through an oral glucose tolerance test, compared to baseline (Chirinos et al., 2014). In addition, the combination therapy led to a greater improvement in triglycerides and insulin sensitivity compared to CPAP alone, but not compared to weight loss alone (Chirinos et al., 2014). In another randomized controlled clinical trial by Georgoulis et al. (Georgoulis et al., 2020
CHAPTER 19
Obstructive Sleep Apnea
INTRODUCTION
DIAGNOSIS
EEG characteristics
Relevance to OSA
Normal amounts
Wake
Low voltage, mixed frequency when eyes are open or alert; α activity (8–13 Hz) while relaxed with eyes closed
Typically increased
15–20% of time in bed
N1
Predominantly low amplitude and relatively fast θ activity (3–7 Hz) accompanied by slow rolling‐type eye movements
Typically increased
4–6%, more in older patients
N2
Sleep spindles (12–14 Hz bursts lasting >0·5 s) and K complexes (large negative EEG wave followed immediately by a slower positive wave) on a background of low voltage, mixed frequency EEG
Typically increased
50–65%, more in older patients
N3
Slow (<2 Hz), high amplitude (> 75 μV) EEG waves for more than half the epoch
Often absent or reduced; associated with improved severity or absence of OSA when present
15–20%, less in older patients
REM
Low voltage, mixed frequency EEG with periodic runs of sawtooth waves accompanied by irregular movements of both eyes and low muscle tone
Often absent or reduced; often accompanied by worsening of respiratory events and more pronounced desaturation than the non‐REM stages
15–20%
Arousals
3–15 s return of waking or faster activity in the EEG; concurrent increase in EMG must be recorded in REM sleep
Commonly occur at the end of respiratory events, but also occur spontaneously or because of other stimuli (e.g., leg movements)
20 per h of sleep
Definition
Apnea
Airflow reduced to less than 10% of baseline for more than 10 s; obstructive if respiratory effort is present, central if no respiratory effort is present
Hypopnea (recommended definition)
Airflow reduced to less than 30% of baseline for more than 10 s, in association with a 4% oxygen desaturation
Hypopnea (alternative definition)
Airflow reduced to less than 50% of baseline for more than 10 s, in association with a 3% oxygen desaturation or an arousal from sleep
Respiratory effort‐related arousal
Sequence of breaths lasting more than 10 s that do not meet the standard hypopnea definition but are characterized by high upper‐airway resistance (snoring and flattened inspiratory nasal pressure signal) and terminate in arousal from sleep
PREVALENCE
PATHOPHYSIOLOGY AND RISK FACTORS
COMPLICATIONS
MANAGEMENT
NUTRITION IN OBSTRUCTIVE SLEEP APNEA
DIET AS A RISK FACTOR FOR OBSTRUCTIVE SLEEP APNEA DEVELOPMENT AND PROGRESSION
DIETARY MODIFICATIONS FOR OBSTRUCTIVE SLEEP APNEA MANAGEMENT
Stay updated, free articles. Join our Telegram channel
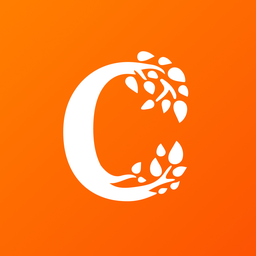
Full access? Get Clinical Tree
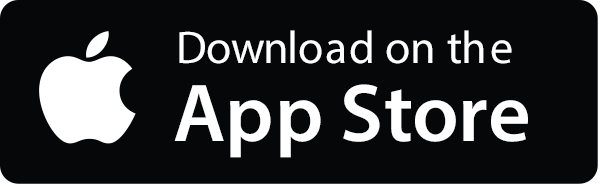
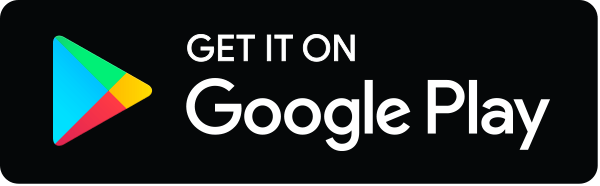