Human genome, the living environment, and lifestyle factors such as nutrition and physical activity are of utmost importance in determining an individual’s lifespan by affecting disease risk and survival (Ekmekcioglu, 2020). Although genetic influence accounts for approximately 20% to 25% of total lifespan, the main part of healthy aging and life expectancy is determined by lifestyle and environmental factors. Improvements in several core lifestyle measures like income, nutrition, education, hygiene, medical therapy, and health care provision have made significant improvement in longevity and quality of life (QOL) (Ekmekcioglu, 2020). A large body of research indicates that nutritional and metabolic factors could also influence health span and longevity by affecting the epigenetic program (Pignatti, Adamo, Stefanelli, Flamigni, & Cetrullo, 2020). For instance, nutritional and dietary factors are postulated to affect DNA methylation by changing the availability of methyl donors and altering the activity of the DNA methyltransferase (DNMT) enzymes as described in Chapter 2. Folate and related B vitamins are key nutrients in 1‐carbon metabolism, the main metabolic pathway that generates methyl groups for DNA methylation, are essential factors that can modify epigenetic age (Pignatti et al., 2020.). Τhe relationship between nutrition and healthy aging is a subject of increased interest among gerontology researchers, since dietary interventions can prevent, or at least delay, the onset of many age‐related chronic conditions (Capurso, 2021). But what qualifies as a healthy diet, in terms of its macro‐ and micronutrients composition, that successfully extends healthy living remains an unanswered question of high priority for human nutrition research (Panagiotou, Michel, Meijer, & Deboer, 2021; Brandhorst & Longo, 2019). Defining a globally healthy diet is not a simple query, since nutritional needs vary substantially by age, sex, disease status, physical activity levels, and cultural norms (Dominguez et al., 2022). Poor nutrition has severe consequences on health that tend to accumulate over a person’s lifespan and negatively influence later stages of life (Bruins, Van Dael, & Eggersdorfer, 2019; Capurso, 2021). As discussed in Chapter 1, according to the WHO, unhealthy diets and physical inactivity are among the main modifiable risk factors that can increase the number of non‐communicable diseases (NCDs) worldwide and consequently, worsen the global burden of disease (Bruins et al., 2019). Western‐like lifestyles and easy access to high‐energy, low‐nutrient rich foods are considered a major part of the problem. However, across countries, there is considerable variation in the consumption of nutrients and foods/food groups. For example, based on a study that compared the macro‐ and micronutrient intake in four European countries, as shown in Table 10.1, while there is a significant part of the population that consumes an excess of energy, sugar, saturated fat, and salt, as part of the Western world, there is another significant part that fails to meet the required or adequate intake for a wide range of essential nutrients, e.g. dietary fiber (Bruins et al., 2019; Mertens et al., 2019). Table 10.1 Percentage of adults with nutrient intakes meeting the estimated average requirement (EAR), adequate intake (AI), or exceeding the maximal reference value (MRV). E% = energy intake; MUFA = mono‐unsaturated fatty acids; SFA = saturated fatty acids. The red, orange, yellow, light green, and dark green signals are ≤5%, 6–35%, 36–65%, 66–95%, and ≥96% of people meeting the EAR, respectively. Sources: (Adapted from Bruins et al., 2019; Mertens et al., 2019). This evidence aligns with data from other studies in older adults that highlight the broadly inadequate dietary patterns in terms of nutrients and food/food groups in this population compared with the current food‐based recommendations. As depicted in Figure 10.1, most adults aged over 50‐years old fall short on recommendations for the most nutritious food groups, including whole grains, seafood, vegetables, fruits, seeds, nuts, and legumes (Roberts et al., 2021). Nevertheless, there is not an ideal dietary pattern that grants healthy aging for all individuals. However, a continuously growing body of evidence, suggests that plant‐based diets are promising in decreasing all‐cause mortality and furthering life expectancy, especially when compared to the effects that Western dietary patterns have on human health and aging. FIGURE 10.1 Dietary adequacy by age groups. Percentage of adults consuming above, below, or at the recommended intakes for food groups in the 2020–2025 Dietary Guidelines by sex and age group, based on dietary data obtained from the 2007–2010 NHANES. A = whole grains, B = dairy; C = seafood; D = vegetables; E = fruit; F = oils; G = nuts, seeds, soy; H = protein; I = meat, poultry, eggs; J = refined grains; K = SoFAS; SoFAS = solid fats and added sugars. Note: Total vegetables includes beans and peas. Protein excludes beans and peas. Source: (Roberts et al., 2021 / with permission of Oxford University Press). One of the most difficult topics regarding the association between nutrition and longevity is the optimal extent/quantity of (age‐dependent) human protein intake (Pignatti et al., 2020). Οlder adults have higher protein needs because of the anabolic resistance, a phenomenon that limits muscle maintenance and accretion. Anabolic resistance is defined as the inability of an anabolic stimulus (e.g., protein provision, hormonal stimulation, and/or muscle contraction) to stimulate muscle protein synthesis (MPS). It frequently occurs with increasing age and when someone becomes inactive or suffers a critical illness. Therefore, anabolic resistance increases protein needs to offset the elevated metabolism of inflammatory conditions such as heart failure, chronic obstructive pulmonary disease (COPD), or chronic kidney disease (CKD) and undergoing dialysis. In healthy older adults and in a variety of diseases, protein anabolism is related to net protein intake (Deutz et al., 2014). The increased availability of amino acids (AAs), especially essential AAs through increased protein intake, stimulates MPS, by activating the mammalian target of rapamycin (mTOR) signaling pathway in muscle cells, which acts to integrate intracellular and extracellular signals that orchestrate cell growth and metabolism (Figure 10.2) (Olaniyan, O’ Halloran, & McCarthy, 2020; Pignatti et al., 2020; Deutz et al., 2014; Olaniyan et al., 2020). FIGURE 10.2 Overview of the mTOR signaling pathway with the basic features of mTOR involvement in regulating MPS. Amino acids (AAs) enter the muscle from the blood stream. Sestrin2 is involved in sensing and signaling the AA to the Rag GTPases. The interaction of Sestrin2 with GATOR2 inhibits mTORC1 signaling in the absence of leucine. GATOR1 is a Rag GTPase‐activating protein that causes RagA/B to switch to an inactive form containing GDP, which inactivates mTORC1 in the absence of AAs. GATOR1 thus inhibits mTORC1. In the presence of AAs, GATOR2 activates RagA/B and inhibits GATOR1, which promotes activation of mTORC1. Rheb is an essential and potent kinase activator of mTORC1. Activation of mTORC1 causes phosphorylation of S6K1 and 4E‐BP1, which promote protein synthesis by activating ribosomal protein S6 and causing the release of el4F4, the translation initiation factor. Source: (Olaniyan et al., 2020 / with permission of Cambridge University Press). There are many reasons why older adults fail to consume enough protein. This includes a genetic predisposition to low appetite, physiological changes such as impaired sense of smell, taste, and vision, poor oral health, inadequate digestion, insulin and anabolic resistance, inflammation, decreased insulin‐like growth factor IGF‐I levels. Medical conditions that lead to age‐ and disease‐associated anorexia, physical pain and mental disabilities that limit shopping and food preparation and food insecurity due to financial and social limitations are also considered possible reasons of low protein intake in older adults. (Deutz et al., 2014; Pilgrim, Robinson, Sayer, & Roberts, 2015; Olaniyan et al., 2020; Lonnie et al., 2018). Additionally, individuals with low physical activity levels have decreased rates of nitrogen retention and therefore, have increased protein requirements to maintain muscle mass tissue (Lonnie et al., 2018). Whatever the reason is, meeting protein needs on a daily basis is necessary for older individuals in order to compensate the age‐related losses in skeletal muscle mass and strength as well as for maintaining physical functionality, and independence. Moreover, adequate protein is needed for preventing common clinical conditions that affect their QOL such as sarcopenia, frailty, or loss of balance (Ortolá, Struijk, García‐Esquinas, Rodríguez‐Artalejo, & López‐García, 2020) (Figure 10.3). According to the existing recommendations, protein intake in older adults should be at least 1 g per kg of body weight per day. The amount should be individually adjusted according to nutritional status, physical activity levels, disease status, and tolerance. The traditional recommendation for protein intake, that is 0.8 g/kg body of weight per day for all adults, is outdated for older persons. Instead, daily amounts of 1.0–1.2 g/kg of body weight is suggested for healthy older adults by several expert groups for its beneficial effects on muscle mass and strength, physical function, hip fracture rates, and frailty (Volkert et al., 2019; Ortolá et al., 2020). In case of illness, protein requirements may be further increased due to inflammation (including inflamm‐aging), infections, and wounds, however, by how much is difficult to assess. Daily amounts of 1.2–1.5 g/kg of body weight have been also suggested for older adults with acute or chronic illness and up to 2.0 g/kg of body weight per day for severe illness, injury, or malnutrition (Volkert et al., 2019). FIGURE 10.3 Protein status: factors that lead to lower protein intake in older persons. Source: (Deutz et al., 2014 / with permission of Elsevier). Although most studies agree on the elevated protein needs of older adults, there is also a non‐negligible number of studies supporting a positive correlation between low‐protein diets (<20% protein‐derived consumed calories) and lower rates of aging‐related diseases. Prospective and randomized clinical trials (RCTs) demonstrate that low‐protein diets in humans enhance metabolic health, promote lean physical appearances, lower blood glucose, and decrease the risk of developing diabetes (Brandhorst & Longo, 2019). As mentioned in Chapter 8, observational studies conducted in so‐called “blue zones” (populations with exceptional longevity rates), indicate that plant‐based, low‐protein dietary patterns with an emphasis on fruit, vegetable, and nut intake are preferred (Chrysohoou et al., 2016; Brandhorst & Longo, 2019; Appel, 2008). The hypothesis is that lower protein intake results in lower activity of the growth hormone (GH)/ insulin‐like growth factor 1 (IGF‐1) axis, which plays a protective role against the (early) onset of age‐related pathologies and aging itself. Cohort studies suggest a positive correlation between high animal‐based protein and fat as well as low carbohydrate consumption with higher mortality (Susan, 2007; Brandhorst & Longo, 2019). But most of these findings fail to remain statistically important when controlled for sex, age, and age group. For instance, when considering men and women more than 50‐years old in the NHANES dataset, a positive correlation between protein intake and increased mortality was not supported (Levine et al., 2014; Brandhorst & Longo, 2019). In fact, individuals older than 65‐years old who consumed a high‐protein diet had a 28% reduction in all‐cause mortality and a 60% reduction in cancer mortality compared with low‐protein diets irrespectively of fat, carbohydrate intake or the protein source (Susan, 2007; Brandhorst & Longo, 2019). Overall, low‐protein intake in humans may be beneficial for those 50–65‐years of age but detrimental at older ages. Nonetheless, more relevant nutritional studies are necessary to have a better understanding of both the benefits and risks of different levels of protein intake during the human lifespan (Pignatti et al., 2020). Protein quality is determined by its AA composition/profile (especially leucine) and digestibility or bioavailability and has a significant impact on protein metabolism. It affects the contribution of a protein to increase the MPS following consumption. Protein digestibility and absorption kinetics are independent predictors of postprandial MPS. Protein digestibility relates to the amount of dietary protein that is effectively digested and absorbed in a form acceptable for body protein synthesis. It is influenced by protein structure, the food matrix in which a protein is contained, the presence of anti‐nutritional factors (factors that can reduce the bioavailability of macro and micronutrients), and the processing method applied to a food. The digestion speed of a protein also influences postprandial protein retention and accretion. Slowly digested proteins, such as casein, are associated with slower AA absorption kinetics, which affects whole‐body protein anabolism by promoting protein deposition (Olaniyan et al., 2020). Proteins obtained after consuming animal products such as eggs, dairy, and meat, are of high nutritional value and therefore, highly digestible (>90%) (Olaniyan et al., 2020). Overall, animal‐based foods are recognized as superior protein sources due to their complete AA composition (including essential AAs), which are more bioavailable and more easily digested, compared to plant‐based sources (Lonnie et al., 2018). Once they become bioavailable, these AAs can help increase postprandial MPS (Olaniyan et al., 2020). Leucine is the AA with an important role in MPS. Animal‐based foods high in leucine, including chicken, lean beef, pork, and fish serve as a potent anabolic signals (Paproski, Finello, Murillo, & Mandel, 2019). Leucine is correlated to increased muscle mass, strength, and preventing age‐induced muscle deterioration through activating the mTOR pathway (Paproski et al., 2019; Olaniyan et al., 2020). Consequently, leucine supplementation is used to prevent further reduction and loss of lean mass in middle‐aged adults (52±1 years) during periods of bed rest. According to the recommendations of the international study group to review dietary protein needs with aging (PROT‐AGE study group), 2.5–2.8 g of leucine per meal is sufficient to reach the anabolic threshold and optimize MPS (Lonnie et al., 2018). Plant protein sources (such as oat, pea, potato) exhibit digestibility values ranging from 45% to 80% (Olaniyan et al., 2020). Studies involving plant‐based proteins prove that they can instigate MPS in levels similar to dairy proteins, but only when ingested in amounts that largely exceed the RDA. This is probably because most plant‐based proteins have a lower leucine content of 6% to 8%, compared to animal proteins with 8.5% to 10%, and thus, when consumed in low doses, do not increase MPS to a comparable extent. In general, plants contain a lower content of leucine (<8% of total protein) in comparison to animal‐based foods (8–14%). However, some plants are still a relatively good source if consumed in larger volumes: dried seaweed (4.95 g/100 g), dry‐roasted soybeans (3.22/100 g), roasted pumpkin seeds (2.39 g/100 g), dry‐roasted peanuts (1.53 g/100 g), and cooked lentils (1.29 g/1 cup). Overall, when matched by leucine content or protein quantity, MPS rates do not differ between plant and animal proteins (Olaniyan et al., 2020) (Morgan et al., 2021). While protein content and AA composition vary between plant species, in general, plant‐based foods have an incomplete AA profile, which in turn obstructs the MPS process (Olaniyan et al., 2020; Morgan et al., 2021; Lonnie et al., 2018). For example protein from legumes is limited in methionine and cysteine; cereals in lysine and tryptophan; vegetables, nuts, and seeds in methionine, cysteine, lysine, threonine; and seaweed in histidine, lysine (Lonnie et al., 2018). However, plant‐based food sources of protein, such as lentils, legumes, and cereals, if combined together (meaning to eat 2 or more of these incomplete proteins at the same time), they form a complementary protein—a protein that contains all the essential amino acids. Nevertheless, plant proteins, particularly soy and wheat, have a lower potential to initiate MPS because their AAs are more easily turned into urea due to essential AA insufficiency. The increased conversion of AAs to urea means that fewer plant‐derived AAs become available in the systemic circulation, resulting in lower postprandial availability of AAs to stimulate MPS (Olaniyan et al., 2020). Last but not least, lower absorption rates of plant‐based proteins can be attributed to dietary fiber, phytates, tannins, and saponins, which act as anti‐nutrients by blocking protein absorption (Olaniyan et al., 2020; Morgan et al., 2021; Lonnie et al., 2018). While there is not a definite consensus on the dietary requirement for carbohydrates, the European Food Safety Authority and Nordic Nutrition Recommendations currently recommend that 45% to 60% of total energy intake should come from carbohydrates, given that total energy balance conditions are being fulfilled. Accordingly, the UK Scientific Committee on Nutrition (SACN) sets an average intake of carbohydrates that corresponds to 50% of daily energy intake. Although carbohydrates could offer a great option for older adults to increase their energy needs, when needed, both high and low in carbohydrates diets pose a threat to health. More specifically, results from the ARIC (atheroscleoris risk in communities) study that included 15,428 people and a meta‐analysis that pooled ARIC data along with seven multinational prospective studies showed that both low and high carbohydrate consumption are associated with a higher all‐cause mortality. However, a significant number of studies (discussed later in this chapter) indicate that the kind/quality is far more important than the quantity of carbohydrates consumed (Großkopf & Simm, 2020). Free sugars include all mono‐ and di‐saccharides added to foods by the manufacturer, cook, or consumer plus the sugars naturally present in honey, syrups, and unsweetened fruit juices (Kehoe, Walton, & Flynn, 2019). In human cells, the presence of high glucose content negatively affects aging of endothelial progenitor cells and fibroblasts. Elevated glucose enhances various aging‐related phenotypes through activation of the p38 mitogen‐activated protein kinase (MAPK). For instance, the consumption of drinks high in sugar, like sugar‐sweetened soda, appears to increase cell aging by 1.8 years for each 8 oz serving per day (Tucker, 2018). High glucose intakes also induce the downregulation of sirtuins, which reduces forehead box transcription factors (FOXO) activity and accelerates cellular senescence (Capurso, 2021). Lastly, conditions that cause hyperglycemia (namely insulin resistance, DM and others) exacerbate inflammation by increasing the production of reactive oxygen species (ROS) and advanced glycation end products (AGEs) leading to tissue stiffening and reduced enzyme functionality (Capurso, 2021; Großkopf & Simm, 2020). These mechanisms are thought to be at least partially responsible for many degenerative diseases, such as hypertension, diastolic heart failure, kidney dysfunction, cataract formation, dementia, and cognitive decline in general (Großkopf & Simm, 2020). According to the WHO, free sugars intake should not exceed 10% of the total daily personal energy intake. This restriction that can reach up to 5% due to existing moderate evidence that high free sugars intake correlates with an increased risk for developing dental carries and weight management problems (Kehoe et al., 2019). The WHO guideline refers to all adult populations, underscoring the lack of data that refer specifically to older adults. Nevertheless, older individuals should make efforts to cut down on added sugars intake, since high added sugars intake is associated with increased levels of adipose tissue, development of frailty, severe pathology and relative symptoms of Alzheimer’s disease and cardiovascular disease (CVD)‐related mortality (Z. M. Liu, Tse, Chan, Wong, & Wong, 2018; Laclaustra et al., 2018; Valencia, Nagaraj, Osman, Rabinovitch, & Marcinek, 2021; An et al., 2018). Epidemiological evidence indicates that the mean intake of free sugars in this population ranges from 5% of the total energy intake in Spain to 11% in the UK and Netherlands, while only 31% of older people living in the Netherlands adhere to the less than 10% guideline by the WHO (Kehoe et al., 2019). Human health and aging procedures are significantly affected by dietary fiber intake. Data from epidemiological studies indicate that persons who consume higher amounts of fiber than their counterparts tend to live longer and healthier lives. More specifically, fiber consumption is linked to telomere length, which highly correlates with chronological age and the pathophysiology of disease and premature mortality. As age increases, telomeres become shorter by approximately 15 base pairs per year. In relevant studies, subjects who consumed bigger amounts of fiber tended to have longer telomeres. In fact, a 10 g increase in fiber per 1000 kcal equaled an extra 67 base pair telomere length, which can be translated to 4.3 fewer years of biological aging (67 extra base pairs ÷ 15.5 base pairs short per year) (Tucker, 2018). This 10 g increase in fiber intake also reduced the risk of death by 11%. Furthermore, other diseases commonly found in older adults, like heart disease, stroke, T2D, osteoporosis, and breast cancer are also less common among those who consume higher quantities of fiber. This can be attributed to shorter telomere length and to the anti‐inflammatory effect that higher fiber intake have; as fiber intake increases, inflammation and oxidative stress markers tend to decrease (Tucker, 2018). This anti‐inflammatory effect is partially mediated by the improved glucose and glycemic control from significant amounts of fiber (Tucker, 2018). It can also be attributed to the fact that adequate or increased fiber intake helps preferable intestinal microbiota proliferation, which positively affects the evolution of several age‐related diseases and syndromes. A poor in microbiota gut environment is linked to the early onset and progression of neurodegenerative diseases, including dementia and Parkinson’s disease, via mechanisms that collectively form the so‐called “gut‐brain axis”. More research data also suggest that the gut microbiome is involved in the complex mechanisms that lead to age‐related muscle wasting and sarcopenia. The intestinal microbiota from malnourished individuals is deeply disrupted and contributes to wasting through multiple mechanisms, including anabolic resistance, malabsorption, induction of anorexia, and reduced synthesis of vitamins, as depicted in Figure 10.4 (Strasser, Wolters, Weyh, Krüger, & Ticinesi, 2021). Inadequate dietary fiber intake also results in impaired bowel function and constipation, commonly found conditions in older adults, that contribute to increased risk for gastrointestinal disease and adversely affect the QOL (Kehoe et al., 2019). Since dietary fiber may contribute to the normalization of bowel functions, and intake is usually low in geriatric patients, the importance of adequate dietary fiber intake is emphasized. According to the European Society for Clinical Nutrition and Metabolism (ESPEN), 25 g of fiber daily are considered adequate for normal laxation in adults and is regarded as a guiding value for older patients also (Volkert et al., 2019); the UK SACN recommends an average intake of 30 g per day. Mean intakes of dietary fiber are below the current recommendations, as seen in Table 10.2 (Kehoe et al., 2019). Since the link between adequate fiber intake and anti‐aging effects through several mechanisms is more established, attempts to promote balanced diets that focus on sources of fiber should continue to be encouraged. FIGURE 10.4 Hypothetical model of the possible association between microbiota and aging trajectories, based on current knowledge of the association between frailty and microbiota. Healthy aging (top curve) may be associated with the maintenance of microbiota eubiosis (i.e., balance between symbionts and pathobionts) that contributes to the overall fitness of the organism in a virtuous cycle. Aging with frailty, characterized by a general, slow decline of health status and performance following acute illness and exacerbation of chronic diseases with occasional and transitory improvements (middle curve), may be associated with a tendency toward microbiota dysbiosis (i.e., reduced species richness and increased representation of opportunistic pathogens). Disability, following an acute disruptive event or accelerated multi‐morbidity (bottom curve), may be associated with severe microbiota dysbiosis, causing a vicious cycle that sustains illness and further decline of health status. Source: (Strasser et al., 2021 / MDPI / CC BY 4.0). Fat, in the form of free fatty acids and triacylglycerols (triglycerides), is a macronutrient consumed by humans through their everyday diet. Fatty acids are major ingredients of triglycerides, phospholipids, and other complex lipids. Foods contain different amounts of fat and types of fatty acids, and these may be affected by processing, storage, and cooking methods (Calder, 2015). Fat plays a very important role in several bodily functions, including energy storage and release, overall metabolism regulation, lipoprotein and cell membrane formation, hormone production, and mechanical organ protection (Arnett et al., 2019). An overview of the correlation between each main category of fat lipid intake with health outcomes will be followed with a focus on older individuals. Saturated fatty acids (SFAs) are a heterogeneous group of fatty acids that contain only carbon‐to‐carbon single bonds. Examples of foods rich in SFAs are meat, especially red meat and products; butter and butter‐fat; dairy foods like milk, yogurt, and cheese; animal fats such as tallow and lard; and plant oils such as cocoa butter, which is common in chocolate, coconut oil, and palm and palm kernel oils (Astrup et al., 2020). Amounts of individual SFAs vary significantly among these sources. For instance, many plant oils exhibit high concentrations of palmitic acid, as do fish and fish oils, while dairy fats are abundant in pentadecanoic acid and hexadecenoic acid. SFAs are also synthesized de novo in humans using either carbohydrates or AAs as substrates (Calder, 2015). Table 10.2 Summary of mean intakes of macronutrients, free sugars, and dietary fiber in older European adults from National Nutrition Surveys. Source: (Kehoe et al., 2019). SFAs are continuously associated with a higher risk of total and cause‐specific mortality (Arnett et al., 2019). Several medium and long‐chain fatty acids were statistically correlated with elevated transcription factors that upregulate cholesterol, fatty acids, triglycerides, phospholipids, and lipoprotein synthesis as well as inflammation mediators (Calder, 2015; Fritsche, 2015). Additionally, even‐numbered SFAs (lauric, myristic, and palmitic acids) correlate with increased levels of coagulation and insulin resistance (Calder, 2015). Given the high prevalence of CVD and mortality, the American Heart Association (AHA) proposed in its 2013 guidelines to reduce saturated fat consumption for all age groups, including older adults, to better control risk factors such as high levels of low‐density lipoprotein (LDL) cholesterol. This recommendation was based upon studies indicating that when food was supplied to adults in a dietary pattern with a macronutrient composition of 5% to 6% saturated fat, 26% to 27% total fat,15% to 18% protein, and 55% to 59% carbohydrates, LDL cholesterol was lowered by 11–13 mg/d compared with the control diet (14%–15% saturated fat, 34%–38% total fat, 13%–15% protein, and 48%–51% carbohydrate) (Eckel et al., 2014). However, based on several other prospective studies, SFA consumption is not responsible for higher coronary heart disease (CHD) risk and mortality, since replacing them with carbohydrates did not have any relative protective action. Furthermore, several systematic reviews of cohort studies have shown no significant association between saturated fat intake and coronary artery disease or mortality, and some even suggested a lower risk of stroke with higher consumption of saturated fat (De Souza et al., 2015; Siri‐Tarino, 2009; Kang, Yang, & Xiao, 2020; Zhu, Bo, & Liu, 2019). Whereas several reports show no association between an increased intake of SFAs and risk for chronic disease, individuals with higher circulating levels of even‐chain SFA (particularly palmitate) have an increased risk of developing metabolic syndrome, diabetes, CVD, heart failure, and mortality (Astrup et al., 2020). This may be due to the difference between dietary saturated fat and circulating SFAs. Moreover, there are cases in which the presence of SFAs via food consumption amplifies the association of predisposing genetic variants with increased CVD or obesity risk (Astrup et al., 2020). In these cases, the reduction of SFA consumption may be beneficial and therefore worthy of recommendation. Lastly, increased SFA intake is associated with shorter lymphocyte telomere length, which is used as an aging biomarker (Dhillon, Deo, Chua, Thomas, & Fenech, 2021). There is a growing body of literature proving that an adequate consumption of n‐3 poly‐unsaturated fatty acids (PUFAs) can promote several aspects of healthy aging. More specifically, prospective cohort studies show that higher levels of long‐chain, n‐3 PUFAs, but not α‐linolenic acid, are consistently associated with a lower likelihood of unhealthy aging. For each interquartile range, the risk of unhealthy aging was lowered by 15% (95% confidence interval, 6% to 23%) for eicosapentaenoic acid (EPA), 16% (5% to 24%) for docosapentaenoic acid (DPA) and 18% (7% to 28%) for total long‐chain, n‐3 PUFA (Lai et al., 2018). Apart from EPA, all other PUFAs are positively associated with telomere length (Dhillon et al., 2021). Other large prospective studies with older adults, indicate that plasma n‐3 PUFA levels were associated with a 27% reduction in total mortality risk across quintiles, increasing the life expectancy of individuals in the highest quintile by about 2 years (Tessier & Chevalier, 2018). A 5‐week, cross‐over, placebo‐controlled study proved that daily fish oil PUFA (3 g daily) was associated with significantly lower plasma triglyceride levels and systolic blood pressure (Ruxton, Derbyshire, & Toribio‐Mateas, 2016). The lower triglyceride levels occurred through a combination of actions, including the diversion of hepatic fatty acids toward β‐oxidation and away from triacylglycerol synthesis, inhibition of hepatic triacylglycerol synthesis, and downregulation of apolipoprotein B100 synthesis required for very low‐density lipoprotein (VLDL) assembly. EPA and docosahexaenoic acid (DHA) help increase the production of eicosanoids with vasoactive effects, the secretion of aldosterone, the generation of nitric oxide by the endothelium, vascular reactivity, and cardiac hemodynamics (Calder, 2015). Through these effects on the lipid profile, vascular function, inflammation and blood pressure, EPA and DHA reduce the likelihood of atherosclerosis and consequently, the risk of CVD in Western populations (Calder, 2015). Furthermore, adequate quantities of n‐3 PUFA in the long term can significantly support muscle anabolic processes in older adults via reducing pro‐inflammatory cytokines, myosteatosis, mitochondrial ROS emissions and improving insulin sensitivity (Tessier & Chevalier, 2018). Lastly, n3‐PUFAs are shown to reverse age‐related synaptic plasticity changes, promote neurogenesis, and maintain brain structure and functions against inflamm‐aging (Cutuli, 2016). n‐3 PUFAs are essential nutrients since they cannot be synthesized de novo in humans. Generally, n‐3 fatty acids intake falls within the acceptable macronutrient distribution range, which is 0.5% to 2% of energy intake [25]. Although fatty fish are the main and most common source of n‐3 PUFAs, shellfish, seafood, seaweed, flax, soy, rapeseeds, nuts, and certain animal products (for instance, meat and eggs), can also help increase total intake (Calder, 2015). No RDA recommendations exist for n‐3 PUFA; only adequate intake (AI) is established for ALA (1.6 g/day for men and 1.1 g/day for women, aged ≥14 years). However, most expert groups suggest 250–500 mg/day EPA and DHA for cardiovascular health, which translates into about 2 servings (140 g, 5 oz) of fatty fish per week. Although older adults tend to consume more fish that are high in n‐3 PUFA compared to younger adults, their intake remains suboptimal, with a mean of 0.19±0.02 oz/day (Tessier & Chevalier, 2018). This finding comes in accordance with other European surveys which report that there is a general deficit of omega‐3 fatty acids in older adults, with 60.1% of people being at risk of clinical deficiency for a‐linolenic acid while 46.9% risked deficiency for long‐chain omega‐3 PUFA (Ruxton et al., 2016). n‐6 PUFAs are most commonly found in seeds, nuts, and plant‐based oils, which mainly consist of linoleic acid. Margarine‐type spreads, which are also rich in n‐6 PUFA, reflect the fatty acid composition of the parent vegetable oil, although some PUFAs may be lost in the hardening process. Linoleic acid also makes a significant contribution to vegetable oils that are rich in oleic acid (e.g., rapeseed, peanut, and olive oils) or palmitic acid (e.g., palm oil). Over the second half of the twentieth century, there was a move in Western countries to increase the use of vegetable oils for culinary purposes and to replace butter and other animal fats with margarine‐type spreads, resulting in a marked increase in intake of linoleic acid in these populations (Calder, 2015). Human cell membrane phospholipids, especially those found in skin ceramides, contain significant proportions of linoleic acid, which prevents skin integrity breakdown and transdermal water loss (Calder, 2015). Conversely, arachidonic acid is predominantly found in brain tissue as a structural component. In its free form, it acts as cell‐signaling molecule that mediates inflammatory processes via the NF‐κB pathway. Lastly, as a structural component of cell membranes, it is usually promoted as a substrate for eicosanoid synthesis (prostaglandins, thromboxanes, and leukotrienes), which regulate immune response, pain experience, bone turnover, platelet aggregation, blood clotting, smooth muscle contraction, renal function, and tumor cell proliferation. A higher arachidonic acid content of cell membranes creates a procoagulant, pro‐inflammatory, proallergic, and protumor environment equivalent to low‐grade inflammation. However, overall literature findings suggest that arachidonic acid has an inverse, or even lack of association with inflammation markers like C reactive protein and TNF‐α or CHD risk (Calder, 2015). In fact, in human studies, higher plasma levels of n‐6 PUFAs, mainly arachidonic acid, were associated with decreased plasma levels of serum pro‐inflammatory markers, particularly interleukin (IL)‐6 and IL‐1 receptor antagonist, and increased levels of anti‐inflammatory markers, particularly transforming growth factor. When healthy volunteers were given seven times the usual intake of arachidonic acid (i.e., 1.5 g/d) in a 7‐week controlled feeding study, no effects on platelet aggregation, bleeding times, the balance of vasoactive metabolites, serum lipid levels, or immune response were observed (Harris et al., 2009). Higher plasma‐PUFA levels correlate with a lower ratio of total to high‐density lipoprotein (HDL) cholesterol, and epidemiologically, replacing 10% of calories from SFA with n‐6 PUFA is linked to an 18‐mg/dL decrease in LDL cholesterol, greater than that observed in a similar replacement with carbohydrate. These findings confirm an LDL‐lowering effect of omega‐6 PUFA beyond that produced by the removal of SFAs. The favorable effects of linoleic acid on cholesterol levels are well documented and predict significant reductions in CHD risk (Harris et al., 2009; Calder, 2015). Trans fatty acids (TFAs) may be created through metabolic processes such as microbial metabolism in the rumen, industrial procedures (for instance during biohydrogenation to harden oils into spreads), or cooking practices like using vegetable oils at high temperatures for frying. The most well‐studied TFAs, in the context of human health, are elaidic acid (trans‐18:1‐n‐9), trans vaccenic acid (trans‐18:1‐n‐11), cis‐9, trans‐11 conjugated linoleic acid (CLA), and trans‐10, cis‐12 CLA. Ruminant milk and meat are sources of trans vaccenic acid and cis‐9, trans‐11 CLA, while the main sources of elaidic acid and trans‐10, cis‐12 CLA are industrially processed oils (Calder, 2015). TFAs bear some resemblances to SFAs, as far as their physical properties are concerned. As a result, TFA incorporation into cell membranes makes the latter more rigid, negatively affecting membrane‐protein functionality and interactivity with lipid raft formations, which lowers the quality of cell signaling. Results from a meta‐analysis of RCTs show that trans‐18:1 fatty acids can raise LDL cholesterol to similar levels as myristic and palmitic acid while decreasing total HDL‐cholesterol levels. The latter observation is quite impressive, since no other lipid molecule has demonstrated this harmful potential (Gayet‐Boyer et al., 2014). TFAs also appear to increase the levels of several inflammatory cytokines, but are not associated with elevated blood pressure or coagulation (Calder, 2015). TFA consumption affects unfavorably the risk of developing CVD and is associated with a higher all‐cause mortality (Calder, 2015; Arnett et al., 2019). Due to their negative repercussions on human health, public health providers suggest abstaining from TFA consumption. Since partially hydrogenated oils are optional food additives, their elimination has been a public health priority (Arnett et al., 2019).
CHAPTER 10
The Role of Plant‐based Diets on Healthy Aging
INTRODUCTION
% Meeting EAR or AI
EAR or AI
Denmark n= 2025 people
Czech Republic n = 1869 people
Italy n = 2831 people
France n = 2624 people
Protein, g/d
0.66 g/kg BW
84%
88%
99%
98%
MUFA, E\%
10–20 E%
69%
92%
75%
77%
Dietary fiber, g/d
25
19%
4%
12%
9%
% exceeding MRV
MRV
SFA, E%
<10 E%
86%
80%
62%
91%
Added sugar, E%
<10 E%
32%
21%
24%
THE ROLE OF MACRONUTRIENTS IN HEALTHY AGING
PROTEIN
Protein Intake: Quantity
Protein Intake: Quality
CARBOHYDRATES
Free Sugars
Dietary Fiber
FAT INTAKE
Saturated Fat
Country (Reference)
Study name
Study years
Age (years)
Protein (%E)
Fat (%E)
Saturated fat (%E)
MUFA (%E)
PUFA (%E)
CHO (%E)
Free sugar (%E)
Dietary fibre (g/d)
Andorra
Evaluation of the nutritional status of Andorra population
2004–2005
65–75
18
40
13
41
17
Austria
Austrian Nutrition Report
2010–2012
65–80
15
36
16
12
7
45
20
Belgium
Belgian National Food Consumption Survey
2004
60+
17
39
17
14
7
44
Denmark
Danish Dietary Survey
2011–2013
65–75
15
36
15
13
6
41
23
Finland
FINDIET 2012
2012
65–74
17
34
14
12
6
45
23
France
Individual and National Food Consumption Surveys
2006–2007
55–79
17
39
11
14
6
44
19
Germany
National Nutrition Survey II
2005–2007
51–80
15
36
15
12
5
47
26
Hungary
Hungarian Dietary Survey 2009
2009
60+
15
38
11
46
22
Iceland
The Diet of lcelanders 2010–2011‐A national dietary survey
2010–2011
61–80
19
38
16
39
16
Ireland
National Adult Nutrition Survey
2008–2010
65+
34
14
12
6
Italy
Italian National Food Consumption Survey
2005–2006
65+
16
34
11
17
4
46
20
Lithuania
Actual nutrition and nutrition habits of adults and elderly of Lithuania
2013–2014
65–75
15
40
12
47
15
Netherlands
Dutch National Food Consumption Survey‐Older Adults
2010–2012
70+
16
34
13
11
7
43
11
21
Norway
Norkost3
2010–2011
60–70
18
35
14
12
6
42
25
Portugal
National Food and Physical Activity Survey
2015–2016
65+
20
29
10
12
5
48
20
Spain
Anthropometry, Intake and Energy Balance in Spain
2013
65+
20
29
10
12
5
48
20
Sweden
Riksmaten 2010–2011 Food and Nutrition Among Adults in Sweden
2010–2011
65–80
17
35
11
17
6
40
5
15
UK
National Diet and Nutrition Survey
2014/15–2015/16
65+
17
34
13
46
11
17
Unsaturated Fatty Acids: Omega‐3
Unsaturated Fatty Acids: Omega‐6
Unsaturated Fatty Acids: Trans Fatty Acids
Fat Intake in Older Adults
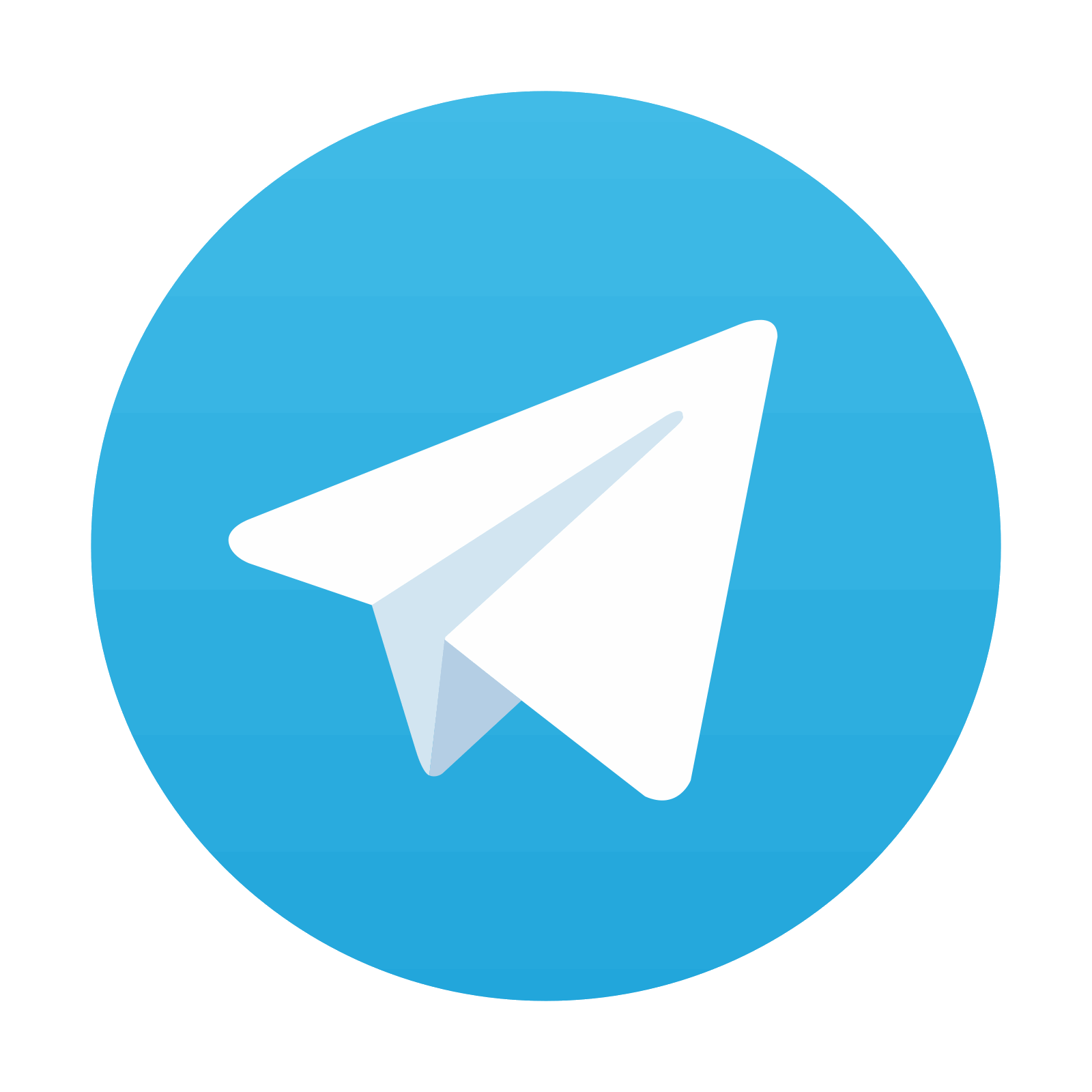
Stay updated, free articles. Join our Telegram channel
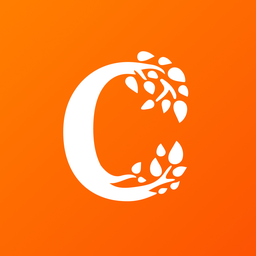
Full access? Get Clinical Tree
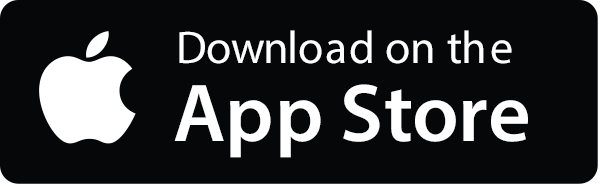
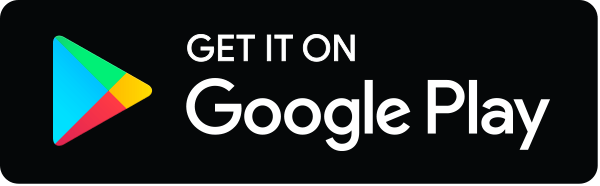